After a first generalized focus on carbon and a second on biodiversity, it looks like water will be the next guest on the podium. A United Nations conference on water was held in New York at the end of March 2023 (the last one was held 50 years ago) to mark World Water Day. The French government launched its National Water Plan in early 2023. And the number of water-related events and trade fairs organized in 2023 has been countless.
With the exceptional El-Nino phenomenon predicted (with a very high probability of occurrence) for the start of the school year in September 2023, it’s certain that the subject won’t be off the agenda any time soon.
In France, the impressive droughts of mid-2022 have finally put everyone in agreement. We’re starting to have a serious problem… as evidenced by the prefectoral decrees in almost all French departments and the delivery of bottled water to several communes in the summer of 2022. Yes, the trouble starts at home. It’s not for lack of warning. The state of the water tables in May 2023 is fairly critical in some French localities, particularly around the Mediterranean, even though the absence of rain in February and March foreshadowed far more terrifying droughts.
But are we really legitimate in France to talk about water scarcity – and by that I mean really scarcity? We don’t really know what water scarcity means. We’ve barely begun to scratch the surface.
Agriculture is by far the biggest consumer of water for crop irrigation. In France, however, only 7% of cultivated land is irrigated in 2020, and this irrigation is fairly unevenly distributed across the country, both spatially and from a crop point of view (certain crops such as corn, soy and potatoes are often irrigated). Clearly, water management is not just about irrigation.
Digital tools are one of many solutions for improving irrigation management and water use efficiency in agriculture. In particular, these digital tools can :
- support the observation and measurement of water in soil, plant and atmospheric compartments (knowledge of the resource), and on agricultural practices (knowledge of practices and their impacts)
- support irrigation management through a wide range of modeling and decision-making tools (optimization of plot irrigation systems and network management)
- support water sharing and governance at different spatial scales (dissemination of information and support for concerted action)
At present, the vast majority of these digital tools are used to support irrigation. This is obviously a very good thing, given the demands we have to make on this scarce resource, but once again, the amount of irrigated cultivated surface remains a minority compared to the amount of non-irrigated cultivated surface.
This dossier on water also has close links with two previous dossiers, on carbon storage in agricultural soils, and nitrogen fertilization management.
This dossier on water is also an opportunity to capitalize on all the knowledge that is beginning to be capitalized on the directory of digital tools for agriculture. In addition to serving as a collaborative watch, this platform is now being used to take a step back from existing digital tools and identify trends.
As usual, for readers of the blog, this article is the result of video interviews with industry players (whose names you’ll find at the end of the article), whom I’d like to thank for taking the time to talk to me. A number of scientific articles, technical reports, websites and webinars have been used to supplement the feedback from the interviews.
Enjoy your reading!
Soutenez Agriculture et numérique – Blog Aspexit sur Tipeee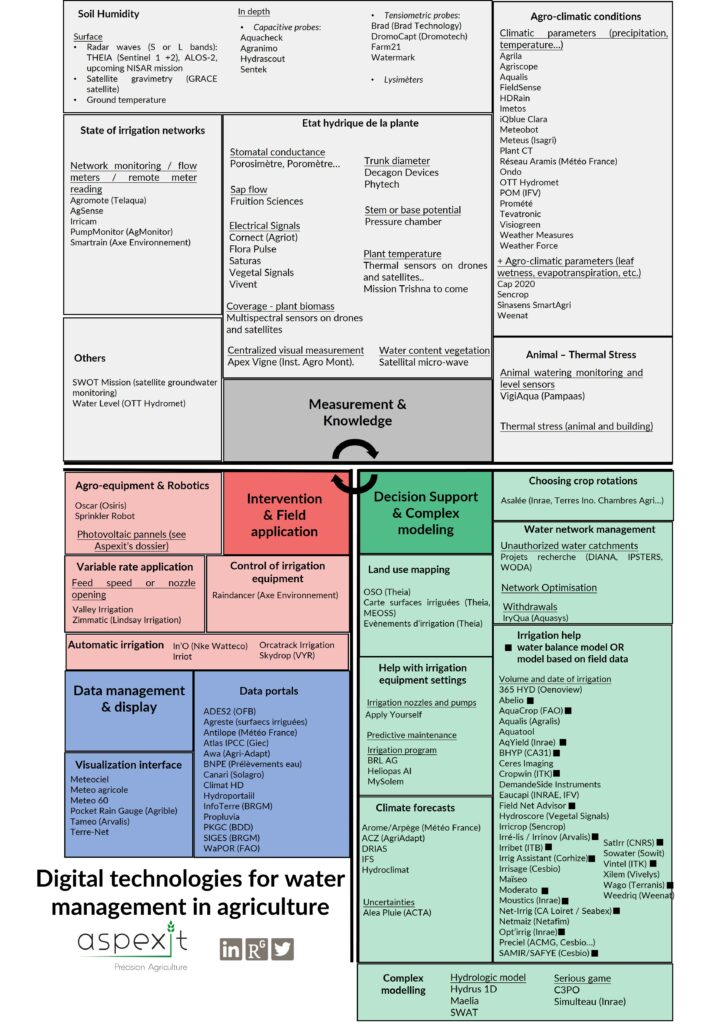
IMPORTANT PREAMBLE
As usual, I’d like to stress that I come to this subject of water with a great deal of humility. I’m an agronomist by training – so I’m obviously particularly sensitive to the subject – but I’m neither a hydrologist nor a hydrogeologist, and even less a climatologist. What I bring to the table is my ability to step back and synthesize what I’ve read and discussed with professionals in the field.
This dossier focuses on water resources from a quantitative point of view. The qualitative aspect is of course also discussed, but to a much lesser extent. As a figure is worth a thousand words, you won’t be surprised to see many maps, diagrams and graphs in this dossier, certainly many more than usual. In view of the very recent concern about the coming water shortage, there is an absolutely gigantic quantity of resources of all kinds in the water field at the moment, all set against a rather muscular backdrop of current events. This dossier on water comes at just the right time.
Let me finish by reminding you that I write popularization dossiers, not scientific articles (although I have written some in the past). Nonetheless, these dossiers are thoroughly researched. They are a synthesis (sometimes only slightly revised) of what I have read and/or heard from my interviewees. For me, popularization is not an oversimplification of reality, but a way of making science more accessible. I try to make this work as objective as possible, even if I do remain committed to my writing.
Please keep this in mind as you read this work!
Water as a first step
A few orders of magnitude
On a global scale, there is no shortage of water – in fact, it accounts for a vast majority of the available surface area.
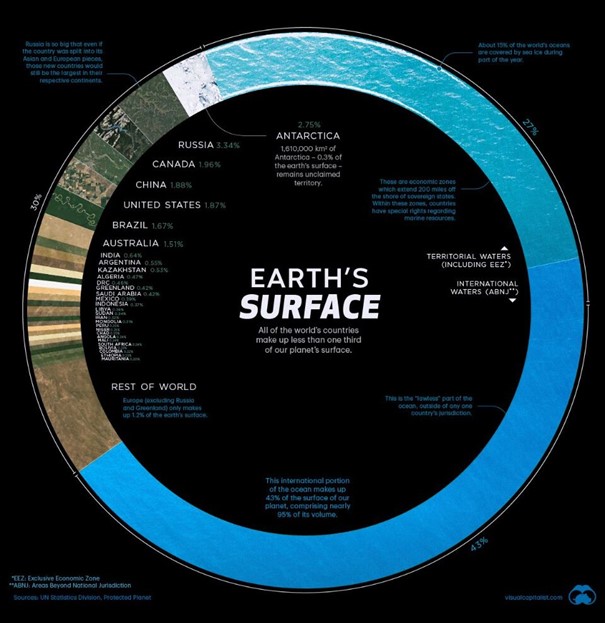
Figure 1: Distribution of water on the Earth’s surface. Sources: UN Statistics Division.
But almost all of this water – 97% – is actually salty (Fig. 1). Only 3% of this water is classified as freshwater. But that doesn’t mean it’s available for our uses. Three-quarters of this 3% of freshwater is found in glaciers. Nearly a quarter of the remainder is present in the soil (in the ground directly or in the water tables – those large pockets of water in the subsoil) and will be used in part by plants to grow if they manage to recover it – more on this later. A tiny part, 1% (we’re talking about 1% of the previous 3%, i.e. 0.03% of the water available on earth), is present in lakes (Figure 2), rivers or in the atmosphere (and will eventually fall as rain).
Unsurprisingly, this water is unevenly distributed across the world – both hydro-geologically (as a natural presence) and anthropogenically (infrastructures in place, control over water, wars over access to water, etc.).
Water can be stored. It is naturally stored in four main natural reservoirs:
- Snow, whose melting generates runoff long after it has fallen, helps sustain the summer flows of the Rhine, Rhône and Garonne rivers. More generally, the cryosphere (glaciers, snow cover, ice caps and, where applicable, permafrost) is the world’s largest natural reservoir of freshwater. It is essential for the vast majority of our uses. Mountains can be considered natural water towers, as they are the source of many rivers and streams.
- The soil, with its large surface area, is a natural water reservoir, and is even the primary source of water for vegetation.
- Groundwater, which can store very large volumes of water, helping to supply rivers with water.
- Natural lakes.
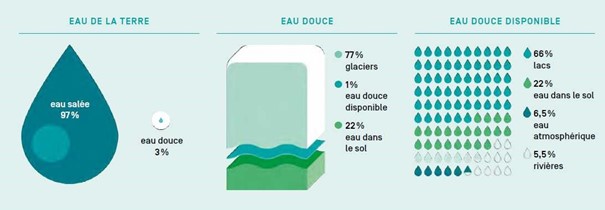
Figure 2: Distribution of water on earth. Source: Inrae (2022).
French geology is made up of a wide variety of rock types, resulting in very different types of aquifer, from sedimentary basins to alluvial plains, limestone and crystalline rocks. There are several thousand aquifers in mainland France, varying greatly in size (the largest being the Beauce and Rhine aquifers), 650 of which are significant enough to be monitored by piezometers. In France, two-thirds of the water abstracted for drinking water supply comes from underground sources. Groundwater resources also account for 31% of industrial water supply and 37% of total agricultural water use.
Aquifers provide a number of services, including :
- Production of quality water in quantity and distributed water because the aquifers are distributed throughout the territory. It’s a bit like a water distribution network provided by nature, unlike surface water, which is only available from the river system.
- Water production and supply to groundwater-dependent aquatic ecosystems. Groundwater-dependent ecosystems in wetlands, lakes, lagoons, etc. depend on the aquifer that feeds them.
- Ability, during rainy periods, to reduce flooding by infiltrating a large part of the water that could run off (a kind of flood regulation service)
Groundwater, like surface water, knows no borders. 592 transboundary aquifers have been identified, including 226 transboundary groundwater bodies. As in the case of national law, international water law initially focused on surface waters. Whatever the size of the aquifer, whether national or extending across borders, national law is of the utmost importance, as it is at this scale that water is managed. In other words, even in the case of transboundary water, water is still managed by national law, and its provisions must enable international law to be applied, and what may be decided by the management commission or joint authority if one exists.
The subject of groundwater is often overlooked – it’s invisible – and conflicts are rarely publicized. In France, the EFESE structure defined 6 types of ecosystem (forest, agricultural, aquatic, coastal, mountain, urban…) but stopped at the sub-surface. Subsoil and aquifers were not considered. By omitting them, the assumption is that aquifers will not be indirectly impacted by human activities (changes in land use, etc.), with the consequent risk of losing some of the ecosystem services provided by aquifers.
In France, groundwater pollution is a major issue. According to the European Water Framework Directive, around 33% of groundwater bodies were considered to be in good chemical condition and 10% in poor quantitative condition in 2013 (water agencies have produced a new inventory of groundwater with the six SDAGE water development and management master plans 2022-2027). Pollution can be diffuse and widespread (as in the case of nitrates or pesticides) or local, as in the case of industrial sites or wastewater treatment plants where, even if effluent is generally well treated in France, you can still find a little nitrogen, pathogens and micropollutants (drugs, phytos…) if you look hard enough. As these pollutants spread, they are diluted or even transformed and, in any case, do not disappear entirely from our environment. Nevertheless, it should be remembered that France has considerably strengthened its arsenal for controlling pollution of aquatic environments in recent years.
Much groundwater needs no treatment. In France, most of the water towers we see in the countryside are connected to boreholes that extract raw water directly. The managers add a little chlorine to prevent the proliferation of bacteria, and the water distributed through the tap, apart from the added chlorine, is drawn directly from the aquifer.
Water cycles – the great misunderstandings of our time
Water is not a mineral that can be mined in one place and transported anywhere on the planet. Water regularly changes state – solid, liquid and gaseous (ice, snow, atmospheric water, river water, etc.) – and moves between the planet’s three main compartments: the atmosphere, the continents and the oceans. Note that these compartments are interconnected and interdependent – water moves continuously from one to another. And the masses of water displaced are absolutely gigantic. So we speak of water cycles in the sense that water, as it changes state, will at some point return to its initial state, but not necessarily always in the same place or over the same periods of time (Figure 3). Bear in mind that the water cycle is a closed cycle. There are no inputs or losses outside the planet. This notion of a closed cycle can be used to question the so-called “water loss” debate (due to agricultural use, for example).
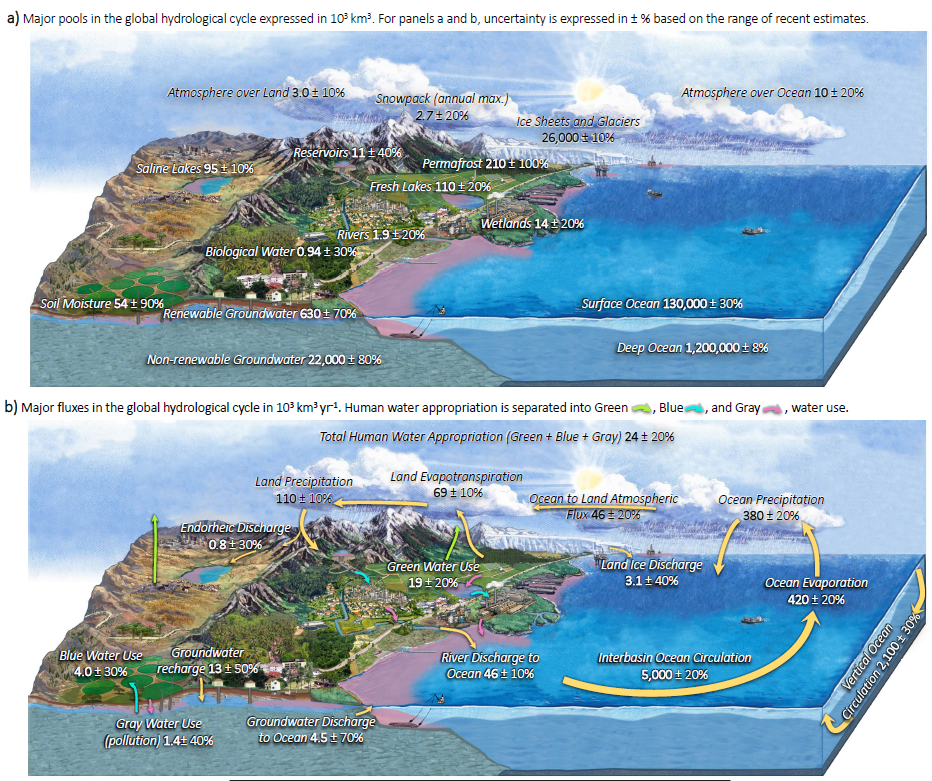
Figure 3. Large and small water cycles. Source: Abbott et al. (2019). Human appropriation of water (the small water cycle) is represented by green, blue and grey water.
The notion of the water cycle may sound familiar to you, having been taught it at an early age. You were happily introduced to the phenomena of evaporation and precipitation, and later in your school career, you linked these terms with changes in the state of water (fusion, liquefaction, sublimation, etc.). The notion of the “large water cycle” refers to the natural water cycle, the one that existed before human intervention. The “small water cycle”, on the other hand, refers to the domesticated water cycle, in which we find all our water catchment, sanitation and supply infrastructures. Some scientists speak of a small water cycle in the sense of a continental or local cycle, as opposed to a global cycle including the oceans, but it’s not clear whether they’re considering human activities here. Just as there is a small water cycle on land, there is also a small water cycle on the seas and oceans. Mutual interactions take place between the different small water cycles, as they occur in space and time over vast areas of different morphologies and surfaces, with varying levels of humidity and surface water. The circulation of water in the small water cycle is therefore partly horizontal (runoff, runoff…), whereas in the large water cycle, vertical movement is certainly the most characteristic (precipitation, evaporation…).
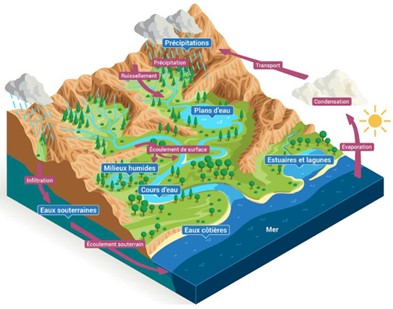
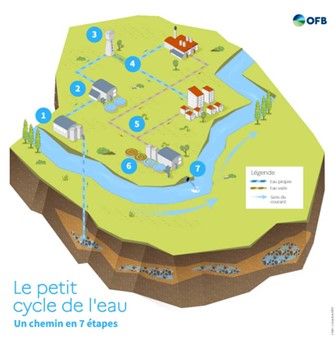
Figure 4: Focus on small and large water cycles.
A number of people are pointing out that there isn’t just one water cycle (the large and small cycles are often considered together), but rather a whole host of them. Hydrologist Emma Haziza, for example, recently spoke on the radio about how there are billions upon billions of water cycles, in the sense that each drop of water has its own path and evolves as it encounters and changes state according to the environments it encounters. Although the image speaks for itself, let’s take a step back and look at a few water cycles that are more or less interwoven.
- The “large” and “small” water cycles I’ve briefly mentioned correspond to the “blue” water cycle, the classic cycle with which we are all familiar.
- Like us, plants transpire to regulate their temperature. This is generally referred to as “evapotranspiration”, because we tend to synthesize both the evaporation of water from the soil and the transpiration of water from plants (water that has previously been absorbed from the soil to plant organs). This water, stored in the soil and plant biomass, is called “green” water. In simple terms, this is the cycle that enables plants to grow.
- Within the green water cycle, we also find the “cloud condensation nucleus”, which works, for example, with mushroom spores (there are other condensation agents besides spores – volatile organic compounds emitted by trees, pollens …). Mushrooms release their spores just before a thunderstorm, and when the rain falls, the venturi effect causes the spores to rise into the clouds in a matter of minutes, forming a condensation nucleus and triggering new rain a few kilometers away.
- The “dew” cycle rings a bell. When the temperature falls below the dew point (for example, when it’s very hot and a tree creates shade, thereby lowering the temperature), in an atmosphere charged with humidity, water from the trees’ evapotranspiration condenses. It’s as if the tree were watering itself, indirectly reproducing the effect of rain.
- Water is also involved in the absolutely fantastic process of photosynthesis. The goal of photosynthesis – the creation of a 6-carbon sugar to provide energy for the plant – requires the presence of 12 water molecules.
To speak of a “small” water cycle (especially on the continents) may give the impression that this cycle only concerns a small quantity of water. This is clearly not the case. Figure 3 shows that continental precipitation flows are greater than water transfers from the ocean to the continent. The Earth generates up to half of its own precipitation from its own terrestrial evaporation, the rest from evaporation over the oceans (Rockstrom et al., 2023).
This capacity could be made possible by the Earth’s “biotic pump” concept (the biotic pump theory has yet to be fully validated). Simply put, continents drink through plants. In a tropical context, a large tree evapo-transpires up to 1,000 liters of water a day to withstand the equatorial heat. It’s a veritable green geyser. The result, for example, is that the Amazon rainforest sends more water into the sky than the Amazon River sends into the Atlantic. A tree is 1 m2 on the ground, but it’s also and above all 150 m2 of leaves that transpire. The world’s great forests send more water into the sky than the entire ocean. According to data from the statistics department of the French Ministry of Energy Transition and Territorial Cohesion, published at the end of 2021, we receive an average of around 510 billion m3 of water every year in metropolitan France, or on the order of just over 900 mm of rain per year. But only 40% of this total, i.e. 210 billion m3, is effective rainfall that goes to groundwater or rivers, with the remainder returning to the atmosphere through evapotranspiration. A reduction in vegetation in a given area thus results in a water cycle that oscillates between droughts and floods.
For the more curious, here are a few explanations to help you better understand the concept of the “biotic pump”.
The water evapo-transpired by the trees in the form of water vapour condenses and forms clouds, often around micro-particles emitted by the trees themselves, with the aim of collecting this future rainwater. As the micro-droplets of water accumulate, they stick together again and again until they become rain. Before bursting, clouds suck moisture from the air below them, creating a depression. At plant level, photosynthesis creates cold by absorbing energy to carry out the photosynthesis process. This cold created at the surface of the leaves pushes water to condense (this is also what we discussed above for the dew cycle). Water thus goes from being a vapor in the air to a liquid on the leaf. The air above becomes lighter, feeding the depression. Clouds on one side, and plants on the other, lighten the air by removing its water, creating a veritable draught that pumps moisture-laden sea air hundreds of kilometers away.
To ensure stable precipitation on the land, we need to ensure that the land evaporates. To simplify things a little, we can say that evapotranspiration of rainwater is the difference between precipitation and runoff (if we disregard the underground accumulation of water). Excessive runoff or runoff over a given area will reduce the amount of water evaporated, and consequently the amount of precipitation.
For some researchers, the correlation between global temperatures and atmospheric CO2 is actually a correlation between vegetation and evapotranspiration, the planet’s biggest energy consumer. Vegetation is primarily responsible for evapotranspiration on earth; collectively, the surface area of leaves is much larger than the surface area of open water. If the sun’s rays fall on a surface well endowed with vegetation and water, most of the solar energy is consumed in evaporation and in latent heat, which does not modify temperatures; the rest is used for photosynthesis, absorbed by the soil, which warms up, and reflected as sensible heat. If the sun’s rays fall on a surface unprotected by plant cover and drainage, most of the solar radiation is converted into sensible heat, resulting in a rise in temperature. Understand that trees, if they receive sufficient water, have an enormous capacity to reduce sensible heat.
A reduction in evapotranspiration (due to deforestation, changes in land use or too much bare soil) would lead to the conversion of short-wave global solar radiation into long-wave emissions and sensible heat. Repeated breaks in the continental water cycle disrupt precipitation-evaporation-condensation processes, releasing thermal radiation and sensible heat several times over. Which came first, the chicken or the egg? It’s in these terms that the opposition arises between, on the one hand, the defenders of the dominant hypothesis in the IPCC reports, who consider that the warming induced by the increase in the concentration of greenhouse gases in the atmosphere is disrupting water cycles, and, on the other hand, some hydrologists who consider that the disruption of hydrological cycles is at the origin of global warming, which is an alteration of the earth’s air-conditioning system and its atmosphere.
In view of the masses of water displaced between all the compartments of our planet, some researchers are calling for a rethinking of our concept of water at the territorial level. Rather than speaking of watersheds or river basins, we should perhaps actually speak of atmospheric basins (Rockstrom et al., 2023). Atmospheric basins (Fig. 5) are made up of precipitation basins, i.e. regions that act as sources of precipitation for others (such as the Central African region), and evaporation basins, which receive atmospheric moisture generated by evaporation from the oceans or continents via neighboring countries.
In a rather unifying article, Ben Abbott and his colleagues take issue with the way water cycles are presented to the general public (Abbott et al. 2019). Very often, the small water cycle is hidden – intentionally or not – leaving us to believe that human activities have an almost insignificant effect on the planet’s major water movements. The authors compare the water cycle to the carbon cycle, where emissions linked to human activity are now widely accepted. It is important to understand, however, that humans undeniably disrupt water cycles in three distinct but interdependent ways.
Firstly, man appropriates water through the use of soil moisture in crop and livestock production (known as green water – we’ll revisit this concept later), water withdrawals (known as blue water) and the water needed to assimilate pollution (some of the water we use may be rendered unusable by pollution and returned to the water cycles). Water withdrawals and consumption for anthropocentric uses are significant (more on this later). Secondly, humans have disturbed around three-quarters of the earth’s ice-free surface through activities such as agriculture, deforestation and the destruction of wetlands. These disturbances modify evapotranspiration, groundwater recharge, river flow and precipitation on a continental scale. Thirdly, climate change – due to its undeniably anthropogenic origins – is disrupting local and global water flow and storage patterns.
Perhaps most importantly, new estimates of human use of green, blue and grey water now total around 24,000 km3 per year. This means that human appropriation of freshwater redistributes the equivalent of half the world’s river flow or twice the world’s groundwater recharge every year. Numerous researchers have testified to the fact that the modelling of major water flows on the scale of different territories (e.g. when using hydrological models) is highly distorted if human activities are not taken into account.
Our imagination of the resource gives us the impression that water availability is largely overrated. By failing to distinguish between saline and freshwater lakes, and between renewable and non-renewable groundwater, we fail to realize that half of the world’s lake volume is saline, and that around 97% of groundwater is non-renewable on centennial timescales (insufficient recharge or unsuitable for human use due to high salinity). And even when we talk about water in quantitative terms, we tend to evoke these total reserve volumes when almost all of it is unavailable. This over-representation is all the more serious given recent evidence that the true volume of renewable groundwater in many regions is less than half of historical estimates – estimates often based on extrapolation. When we talk about water, we are also unclear about the proportion of basins and run-off accessible to man. Less than 10% of annual terrestrial precipitation and 25% of annual river flow are sustainably available for human consumption, and only 1-5% of fresh groundwater is sustainably extractable. Finally, by masking our use of grey water (water pollution), we fail to highlight the fact that human activities have further reduced the small fraction of fresh water still accessible by 30-50%.
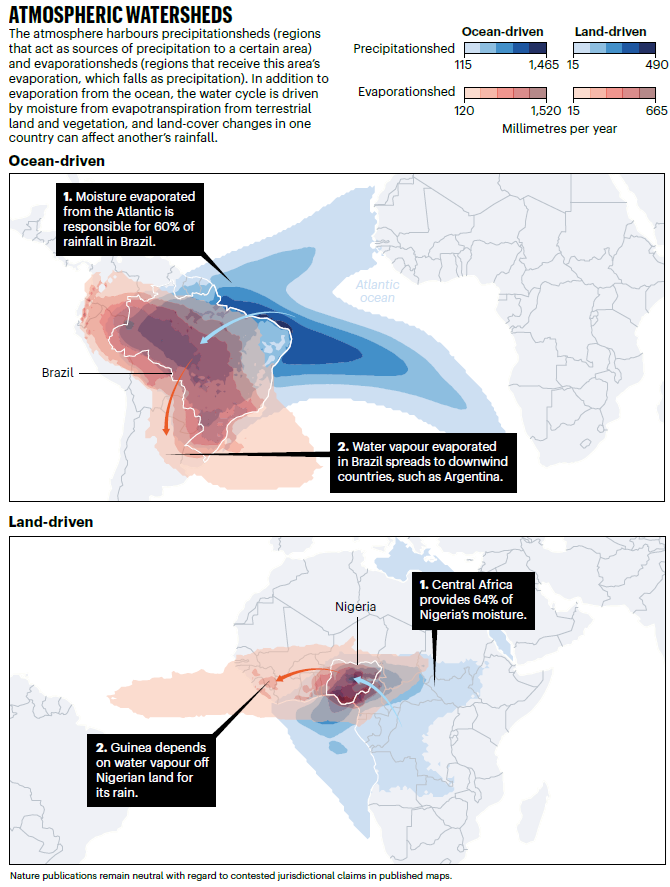
Figure 5. Atmospheric basins.
According to the authors, landscape modifications can alter water supplies in downwind regions, and modify local climates and river flows. For example, deforestation in the Congo Basin reduces rainfall in neighboring countries, and even across the Atlantic in the Amazon. Intensive crop irrigation in India can increase the flow of the Yangtze River in China, thanks to moisture transported in the direction of the wind. Rockstrom et al (2023) add that around 60% of Brazil’s precipitation comes from moisture evaporated from the Atlantic, and that 35% of the moisture comes directly from Brazilian lands, including the Amazon rainforest. Much of this atmospheric humidity remains within the country, trapped by the high Andes. Brazil also exports 25% of its green water to downwind countries such as Argentina, Bolivia and Colombia. Rain from the ocean is evapotranspired by the forests and then re-precipitates, 7 to 8 times in a row, over distances of 500 km each time, in Europe, for example, from the Atlantic coast to the Urals. Without this green conveyor belt, rain would only fall for a few hundred kilometers inland at most.
Significantly, no single country obtains more than half its moisture from within its borders. Even the largest countries therefore depend on evaporation from other regions to maintain their rainfall. Under international law, then, it seems legitimate to ask every country to protect the global water cycle, since any action taken in one place would have repercussions elsewhere on earth.
Our intimate relationship with water, by which I mean the fact that our actions have direct effects on the planet’s water cycles, invites us to “sow” and “cultivate” water (Gallabert et al., 2022). Water cannot simply be considered as a resource that we take and then discard, but as a whole integrated into cycles that go beyond us. It makes no sense simply to talk about water stocks. In view of all that we have described, it seems clear that the notion of flow is paramount.
The disruption of water cycles also allows me to quickly introduce the concept of planetary limits, developed by Swedish researcher Johan Rockstrom and his teams (Fig 6). By May 2023, seven limits had already been exceeded, including those around the freshwater cycle – separated into green and blue water (see the different cycles presented above). Some of the most prominent scientific publications consider only water consumption in determining sustainable planetary limits for freshwater, and others present terrestrial evaporation and transpiration as water losses rather than as the main sources of freshwater for agriculture and ecosystems. In mid-2023, Rockstrom and his teams proposed a revision of their concept of planetary limits to incorporate a strong notion of social justice. These include the “safe” limits, which are those that call into question the resilience of the system when exceeded (these are the ones present in the initial infographic – they have been slightly reworked in the meantime). We also see the “just” limits, which are those at which humans start to suffer significantly (even if this is still tenable for the planet).
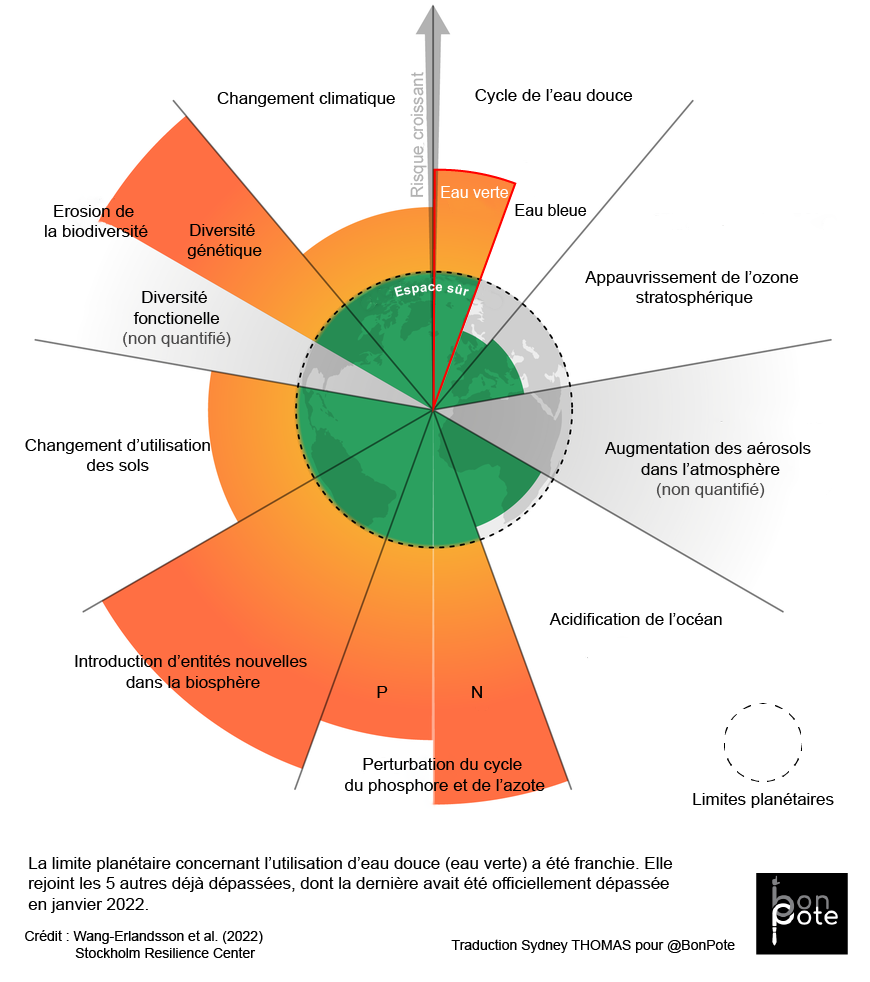
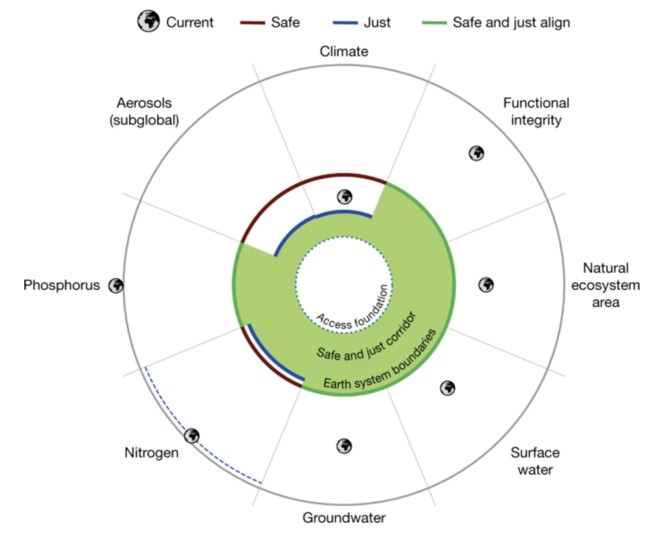
Figure 6. The planetary boundary concepts of Rockstrom and his teams. The bottom infographic is the new version of the proposed planetary boundaries. Source: Safe and just Earth system boundaries (2023)
What can we expect in the years to come?
A downward trend in global freshwater availability
Water has a very strong symbolic value. Both a source of life and death, water can be in short supply or, on the contrary, in excess. This water symbolism can be found in religious texts through the desert (lack of water) or the flood (excess of water).
In France, up until now, our vision has tended to focus on excess water, with the floods and flooding our territory has experienced. We also have a national flood risk management strategy (SNGRI) aimed at better identifying at-risk areas and taking action to reduce the vulnerability of territories, flood risk prevention plans (PPRI) aimed at controlling urban development in flood-prone areas and preserving flood expansion zones, and a competence for managing aquatic environments and flood prevention (GEMAPI) carried out by public establishments for inter-communal cooperation (EPCI), i.e. inter-municipalities.
But since mid-2022, it’s been water scarcity that’s been taking center stage in the French news. And this is perhaps all the more disturbing when, at the same time, certain countries on the front line of the effects of climate deregulation are suffering the ravages of deadly floods (Pakistan in August 2022, for example).
In April 2023, the Copernicus Climate Change Service published a map comparing river flows in Europe at August 2022 with a reference period of 1991-2020 (Figure 7). The map speaks for itself, with the vast majority of flows significantly or exceptionally low. According to them, 2022 was the driest year on record (they haven’t worked on 2023 yet, rest assured), with over 60% of rivers experiencing below-average flows.
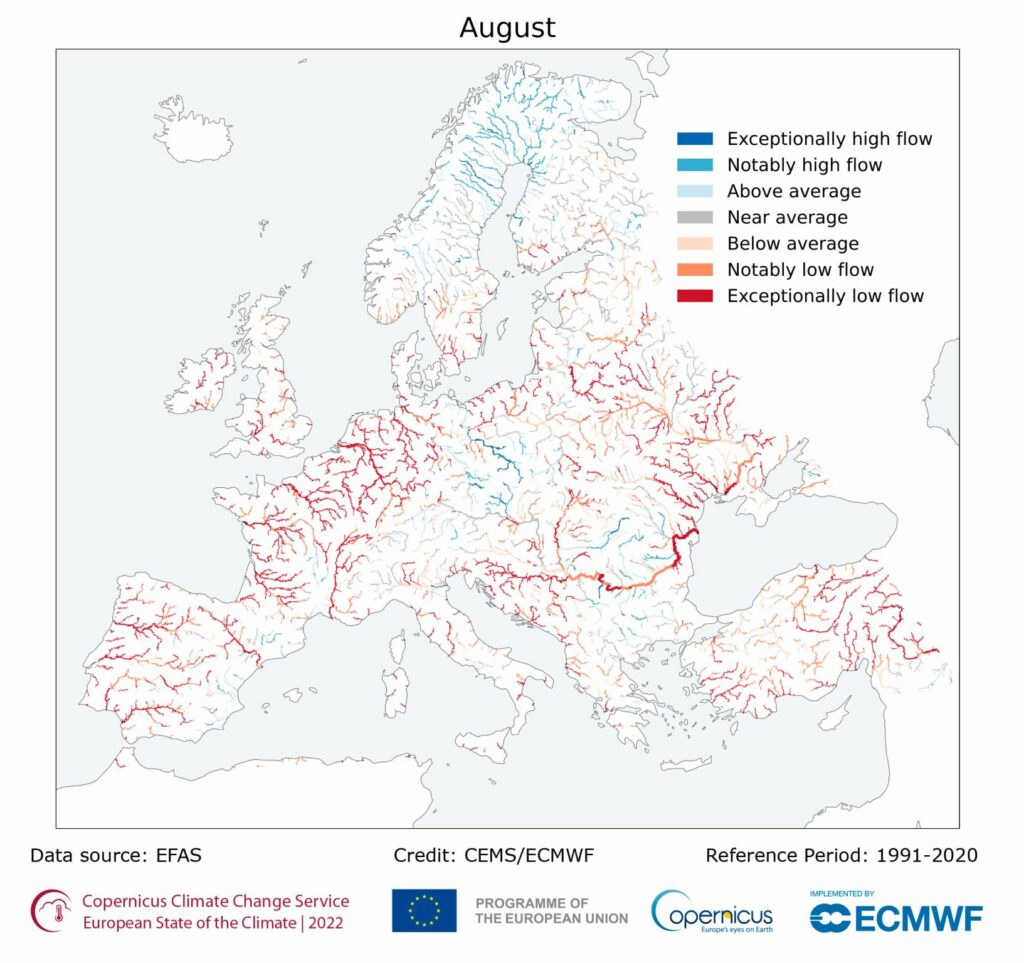
Figure 7. Comparison of river flows in Europe between August 2022 and the 1991-2020 reference period. Source: Copernicus Climate Change Service (2023).
In 2022, the World Meteorological Organization published its report on the state of the world’s water resources (Figure 8). This included a comparative map of average river flows in 2021 compared to a reference period of 1991-2020, a way for them to look at whether, on average, river flows were higher or lower than the temporal average. The authors pointed out that areas where river flow was below or well below normal were twice as large as areas where river flow was above or well above normal.
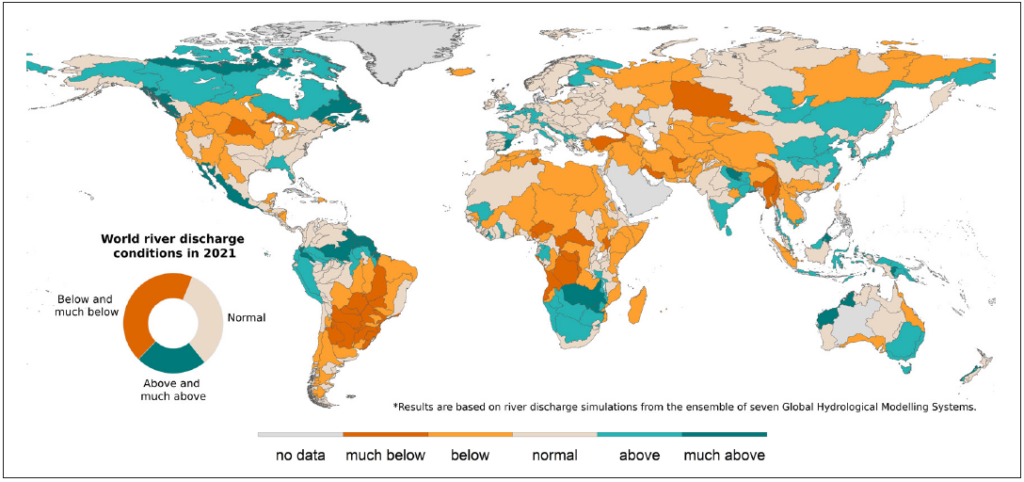
Figure 8. River discharge in 2021 compared with a 1991-2020 reference period. Source: World Meteorological Organization (2022).
Also at global level, an article published in Science in May 2023 showed a trend in the amount of water stored in the world’s largest lakes over the last 3 decades (Yao et al., 2023). Of the nearly 2,000 lakes analyzed (both natural and man-made), more than half would have suffered a dry loss of volume, especially in the world’s arid regions (Figure 9). And the majority of this loss is observed in a minority of lakes (some large lakes have therefore lost a great deal of water). The main reasons put forward for this are sedimentation (sediment leaves less room for water), climate change (higher temperatures leading to increased evaporation, changes in precipitation patterns leading to drought), and human activities (for our various uses – we’ll talk more about this later). The impacts of climate change and sedimentation therefore need to be integrated into a broader approach to the sustainable management of water resources.
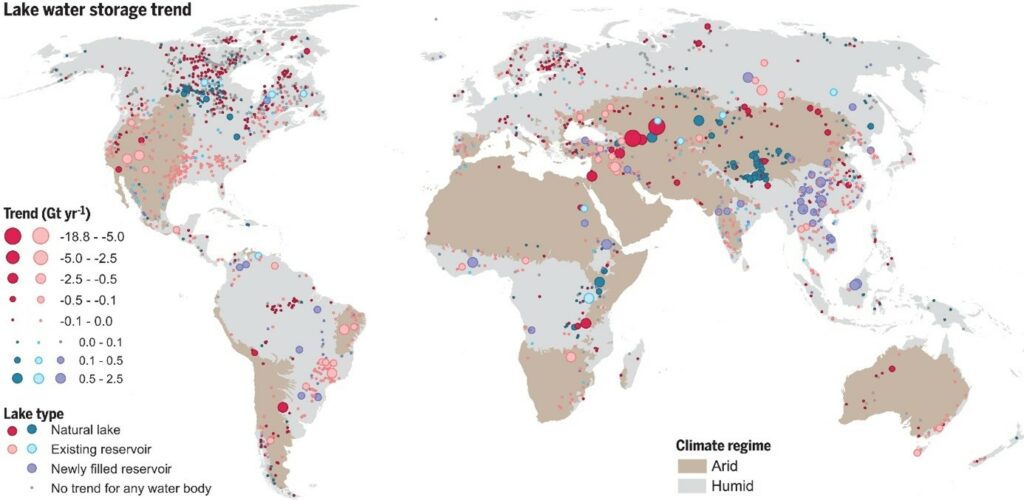
Figure 9. Evolution of water storage in the world’s largest lakes Source: Yao et al. (2023).
The year 2022 was ranked between the fifth and seventh hottest on record, with an average annual temperature 1.15°C above the pre-industrial average of 1850-1900, despite the prevailing “La Niña” weather phenomenon. Scientists fear that by 2023, the return of the “El Nino” phenomenon (whose probability of occurrence is considered very high) could further exacerbate global warming.
The 2008 map below (there is no more recent map to my knowledge) shows the world’s groundwater resources. It’s quite impressive to see that between 1960 and 2010, i.e. in the space of 50 years, groundwater withdrawals in all sectors tripled. It is estimated that some 60-70% of the world’s groundwater is used for agricultural purposes, with the remaining 30-40% divided equally between industrial and urban drinking water supplies.
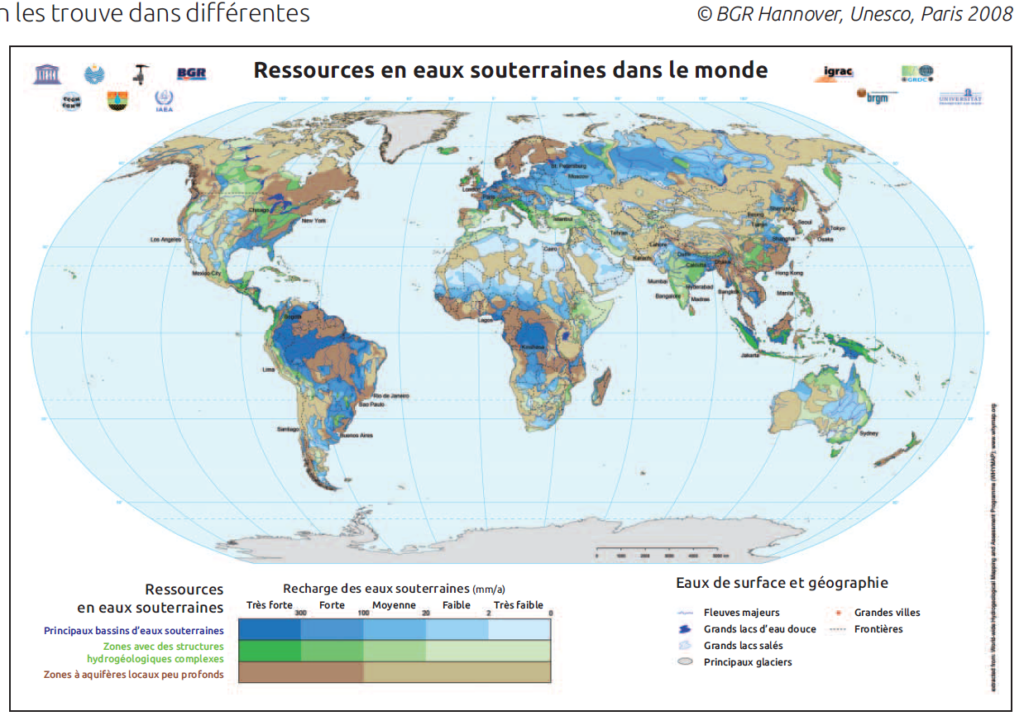
Figure 10. Groundwater resources worldwide. Source: BGR Hannover, 2008
Rather alarming projections in France
There are countless climate projections and forecasts in progress (Aqua 2030, Climator, Garonne 2050, Varennes de l’eau, Explore 2070…). All these projections point to an expected change in precipitation patterns, with a structural reinforcement of water deficits (particularly in summer). The impact on water resources will be marked, with expectations of higher average air temperatures, uncertain precipitation trends, and a significant reduction in average annual river flows (the current maps presented in the previous section speak for themselves). The chronicle of recent years, retraced by the Bulletin national de situation hydrologique (BSH), published monthly under the aegis of the International Office for Water, shows that drought is no longer a one-off phenomenon (as in 1949 and 1950, 1976, 1989-1990 and 2003), but is tending to become recurrent and widespread.
Also note that in early 2021, Météo-France produced new regionalized reference climate projections for France, available on the DRIAS website.
The Explore 2070 study is perhaps the most reliable reference on the evolution of water resources. The project’s results show that average river flow in France is set to fall sharply over the next 30 years, by as much as 50% in the south-west and the Paris Basin. The Explore 2 project, currently underway, will focus on running hydrological models to derive the impact of climate scenarios on future flows in all French rivers. It is important to understand that these projects calculate reconstituted natural flows, i.e. a forecast of the state of resources taking into account climate change scenarios and land use. What will actually be present in the rivers (in relation to the simulation results) will obviously depend on our social choices.
The conclusions of the Explore 2070 report on river levels and groundwater recharge rates expected in France between 2046 and 2065 are rather eloquent, and all point downwards. In particular, we can expect :
- An average drop in groundwater recharge of 10 to 25%, with the Loire basin (25 to 30%) and the South-West (-30 to -50%) showing a marked decline.
- A drop in average annual river flow of between 10 and 40%, particularly for rivers in the Pyrenean foothills and the Seine-Normandy basin (between -10 and -60%).
- A 30% to 60% reduction in summer low flows, which are more severe, longer and earlier: up to -50% drop in minimum monthly flow for the Rhône, -70% for the Seine.
- A reduction in the proportion of precipitation recharging the water table through infiltration or runoff to rivers and lakes, as a result of rainfall variations and evapotranspiration phenomena.
- Increased evapotranspiration, leading to a greater proportion of precipitation being retained in surface soils, to the detriment of groundwater recharge.
- A shortfall of 2 billion m3 of water by 2050 if demand remains stable
- An expected decrease in river flow of -20 to -40% in the Adour-Garonne basin
Climate trends will have a significant effect on both large and small water cycles, modifying snow cover (and the cryosphere in general) through a significant reduction in snow cover and the duration of snow cover, as well as river dynamics (flow rates, infiltration, evaporation), surface and ground water management (quantity and quality) in terms of availability, through a drop in the average level of water tables, supply and sanitation, the fragility of aquatic and wetland ecosystems and biodiversity, or the level of soil dryness and, consequently, agricultural production. The IPCC also points out that all climate scenarios project an increase in the risk of drought linked to soil moisture.
The results of the Explore 2070 and Climator projects may even be underestimated, since they assume that future water shortages will be offset by more irrigation, something which – as recent events show – does not make everyone agree (more on this later). Let’s not forget that irrigation systems are faced with other problems: water scarcity due to the effects of drought and climate change, environmental disturbances, the non-linear nature of plant dynamics, the changing dynamics of weather conditions, and the dynamic uptake of water by plants.
Back underground. Groundwater is already being affected, and will continue to be increasingly so. Ongoing climate deregulation (rising temperatures and falling rainfall) is disrupting water cycles, including in groundwater, and causing a drop in natural recharge rates. Reduced water availability will have an impact on low-flow river flows because, in summer, the water circulating in surface waters is mainly groundwater. This is particularly true of alluvial aquifers and other shallow aquifers connected to surface waters.
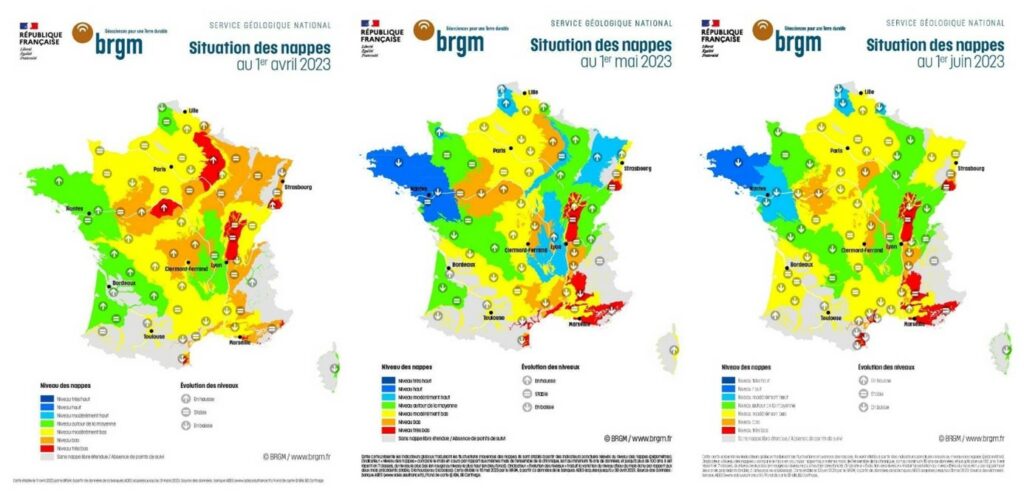
Figure 11. Groundwater situation in France in April and May 2023. Source : BRGM. It had been 𝟏𝟔 𝐦𝐨𝐢𝐬 since we last saw dark blue on the map (that was on January 1, 2022). Levels are mostly down
In terms of groundwater hydrology, the results of the Explore 2070 project in France show, for the 2050-2070 timeframe, an almost general decline in piezometry associated with a decrease in water recharge of between 10 and 25% due to climate change. Two areas are more severely affected: the Loire watershed, with a fall in recharge of between 25 and 30%, and south-western France, with falls of between 30 and 50%. This decline is likely to result in lower low-water flows and longer dry periods. Climate deregulation scenarios can be fed into water flow simulation models (groundwater models) to simulate the impact on groundwater levels and the low-water flows that will reach rivers (and thus assess the duration of these lower flows, working basin by basin…). The main scenario for change is an overall reduction in groundwater abstraction of 20% by 2070.
The lowering of the water table can also threaten wetlands. Maintaining a water table at or near the ground surface for a sufficiently long period each year to allow the survival of aquatic plants is a challenge for some wetlands. For example, the Marais Poitevin is at high risk of negative impacts during droughts due to the pumping of groundwater for corn irrigation.
For coastal aquifers, another issue is saline intrusion. In these aquifers, which are directly connected to the sea at depth, saline intrusion can occur because the denser seawater gradually infiltrates the groundwater. The higher the water table, the more saline intrusion can be postponed. The lowering of water tables as a result of climate change and groundwater pumping will increase the risk of saline intrusion in all coastal aquifers. In France, this is not yet a major issue, but we need to be prepared.
Quantitative imbalances due to pumping higher than natural recharge (which therefore implies a drop in groundwater levels) appear to be less of a concern than qualitative imbalances.
The potential impacts of chronic water scarcity
Without going into too much detail, we can draw some general conclusions about the impacts already present and those to come.
In August 2022, more than 500 French communes found themselves without drinking water and had to rely on tanker trucks for their supplies. While some still see this as a warning sign, in the sense that these supplies were mainly to small communes and not to large conurbations with more robust supply networks, the risk of water shortages is real and affects us directly. In May-June 2023, some French communes are already preparing to reproduce last year’s deliveries…
On closer examination, the lack of water goes far beyond a “simple” supply of drinking water to the population.
With falling river levels and irregular flows, shipping is de facto limited. Rhine levels, for example, were exceptionally low in 2018, blocking goods in the port of Cologne. You may remember blissfully witnessing the blockage of the Suez Canal in March 2021 by a giant container ship. The blockage wasn’t caused by low water levels, but you’d imagine it was. At present, more than one tonne of goods is transported by inland waterway for every inhabitant of the European Union – and we’d like to see this level rise to encourage more carbon-free freight than it is at present.
Hydroelectric dams run at a reduced rate when the flow decreases, with the result that hydroelectricity production falls and industry is affected. And river freight also transports coal to produce energy – and compensate for the reduced output of hydroelectric power stations, which is also limited by lower river flows (perhaps we’re going a bit crazy here). Water levels may also raise questions about their use for cooling nuclear and thermal power plants (more on this later, but bear in mind that, in France, the risk of a water shortage in this respect is a power plant shutdown, not a nuclear risk).
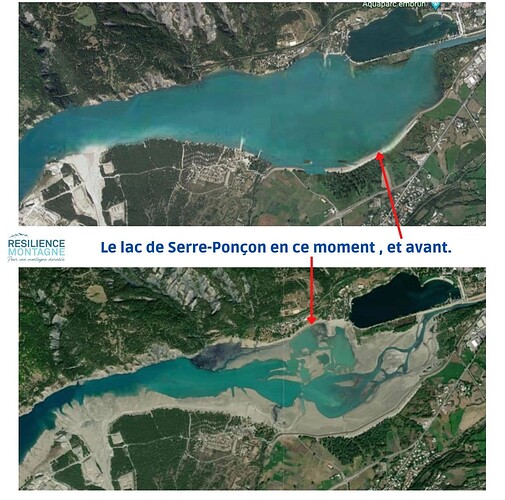
Figure 12. Condition of Lac de Serre-Ponçon (hydroelectric dam) in summer 2022. Source: Resilience Montagne.
A drop in water levels can also result in supply chain disruptions (not necessarily due to river transport problems). The drought that hit Taiwan in 2021 had a major impact on TSMC, which supplied semiconductors to the whole world.
As far as agriculture is concerned, the findings are obviously quite dramatic (and we’ll look at them in more detail later). At the beginning of 2023, Catalan farmers decided to stop sowing alfalfa and corn due to very unfavorable conditions. In Italy, the level of the River Po seems to be calling into question rice irrigation and clam farming. Lower water levels encourage the appearance of sandbanks, but also, and perhaps above all, of salt marshes – i.e. saline upwelling. Rising sea levels (as a direct consequence of global warming) and the pumping of groundwater encourage saltwater to rise ever further (we could also talk about the salting of roads, where salt ends up in the water after rain, but this phenomenon is perhaps negligible). With increasingly brackish water, soils become salinized once the water has been absorbed by plants, or simply through evaporation of water from the soil, significantly affecting agricultural production on these soils. The increase in brackish water could also call for a reconsideration of certain types of production that would simply no longer be practicable. Would it be possible to switch from growing rice to shrimps in water that is too salty?
Less water flowing in rivers means less dilution of pollutants, and therefore, mechanically, greater concentration. Reduced flows also have a dramatic impact on biodiversity, fragmenting environments and preventing the mobility of fish and amphibians, particularly to their spawning grounds. We’ll come back later to the concepts of ecological and biological flows, which have been developed to limit the impact on living organisms and ecosystems in the broadest sense.
The chronic shortage of water will inevitably call for arbitration between different uses. For example, when hydroelectric dam concessions come up for renewal, hydroelectric and agricultural uses are likely to be in competition. The peak irrigation period is not the same as the peak electricity consumption period (i.e. dams have no interest in releasing their source of electricity when people don’t need electricity in summer). Each sector will therefore defend its strategic importance, as well as the availability, or even sanctuary, of sufficient resources, with two differences that penalize agriculture. On the one hand, the irrigator’s uses are linked to a single source of supply (water intake or reservoir), whereas modulations in electricity demand can be supported by a far more flexible system of interchangeable power plants and connected distribution networks. On the other hand, the energy sector may be tempted to optimize its revenues by offloading as little of its storable production capacity as possible, in order to use it when consumption peaks, which are also its tariff peaks.
What impact can we expect for plants?
A plant’s yield potential is the yield it can achieve when water and nutrients are not limited and potential biological stresses are controlled. Under these optimal conditions, only solar radiation, temperature, atmospheric CO2 and the plant’s genetic characteristics have an impact on the length of the growth period and light interception by the plant canopy. This notion of yield potential is theoretical. It is a reference that is considered relevant in irrigated agricultural contexts (since it is assumed that there is no shortage of water) or in humid climates with a sufficient water supply to avoid water deficits (Van Ittersum et al, 2012).
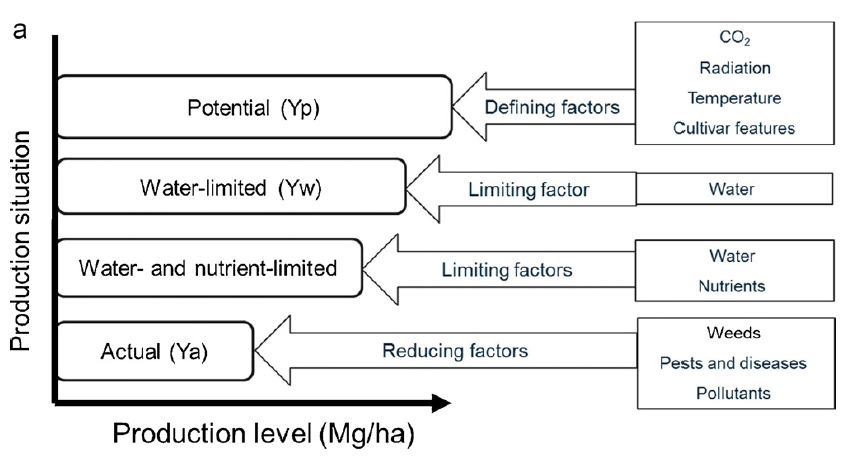
Figure 13. Presentation of different levels of yield potential. Source: Van Ittersum et al (2012)
When water is a limiting factor – as in the case of rain-fed crops, for example – we prefer to speak of yield potential limited by water (and/or limited by nutrients, if these are also in short supply). The assumption here is that soil type and topography have a sufficiently significant impact on crop yield (water retention capacity, rooting depth, runoff intensity). Note that the gap between potential yield and water-limited yield is an indication of the yield gap that could potentially be bridged by irrigation (under similar external conditions).
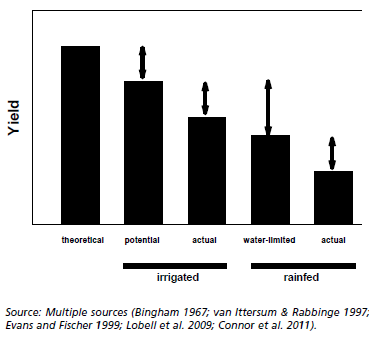
Figure 14. Yield potential under irrigated and rain-fed conditions
In real-life conditions, these theoretical yields are rarely achieved. Yields are in fact affected by a whole host of other biotic factors, such as disease infestation and the presence of pests, as well as abiotic factors (input availability, available agro-equipment, farmer training, etc.). The yield gap is thus the difference between actual yields and those potentially achievable, whether or not there is a shortage of water.
To be meaningful, these notions of yield potential must be estimated for a defined geographical unit and time period. Several methods are available to approach them:
- simulation of potential on a given site using crop growth models. This is certainly the most accurate estimation method, but also the most data-intensive.
- maximum yields or upper quantiles of yield measured, for example, in experimental stations or on farmers’ farms – this is an empirical, observation-based method, where we work with yields actually obtained, but which may also suffer from bias (agro-pedo-climatic conditions may be exceptional in these areas). This method is particularly applicable to rainfed systems and allows us to identify the seasons and conditions that most favor water-limited yields, and thus suggest management strategies and tactics to maximize water productivity across the range of environmental conditions analyzed (Van Ittersum, 2012).
- the use of a “yield vs. water” frontier function [often the French and Schult model] obtained from the observation of relationships between farmers’ yields and water supply. Even if this frontier function does not differentiate between study cases where the water supply is identical but the limiting factors are different (since only water availability and yield obtained are displayed), it can still help determine the presence of limiting factors and has the advantage of covering wide ranges of available water quantity. This method could also be criticized for not taking into account the temporality of water supply, in the sense that certain periods are more critical than others for determining yield. Seasonality and rainfall patterns obviously play a decisive role.
Local approaches are obviously to be preferred wherever possible, but data is not always easy to retrieve. Global approaches, on the other hand, may be limited by a misrepresentation of what is happening in reality. Cropping systems (crop rotation, planting date, cultivar maturity, etc.), which play a major role in yield results, are sometimes not taken into account, or are very heterogeneous in the samples considered.
Insofar as water is clearly a hot topic at the moment, it seems appropriate to also introduce the notion of water “productivity”. The term may seem a little surprising for a resource considered a common good, but it’s a subject at the heart of food production and resource use efficiency. Echoing the productivity of nitrogen use (see nitrogen dossier), water productivity can be simplified to mean the relationship between yield and seasonal water supply (precipitation, irrigation, soil water, etc.). In real-life conditions, the quest for maximum yield is rarely the best strategy, as we may find ourselves in a situation where we implement cultivation practices that are either not economically rational, or too risky in economic and environmental terms.
Climate deregulation will inevitably lead to an increase in plant evapo-transpiration and changes in precipitation patterns, with considerable regional variability. From a relatively macro viewpoint, we can expect additional water deficits in summer, limiting the availability of water in the soil, and even more so in soils with limited water retention capacity. The possible increase in winter precipitation could lead to excess water s producing stresses just as penalizing as summer droughts, with root anoxia leading to lower yields in wet years if adjustments or changes in practices are not implemented.
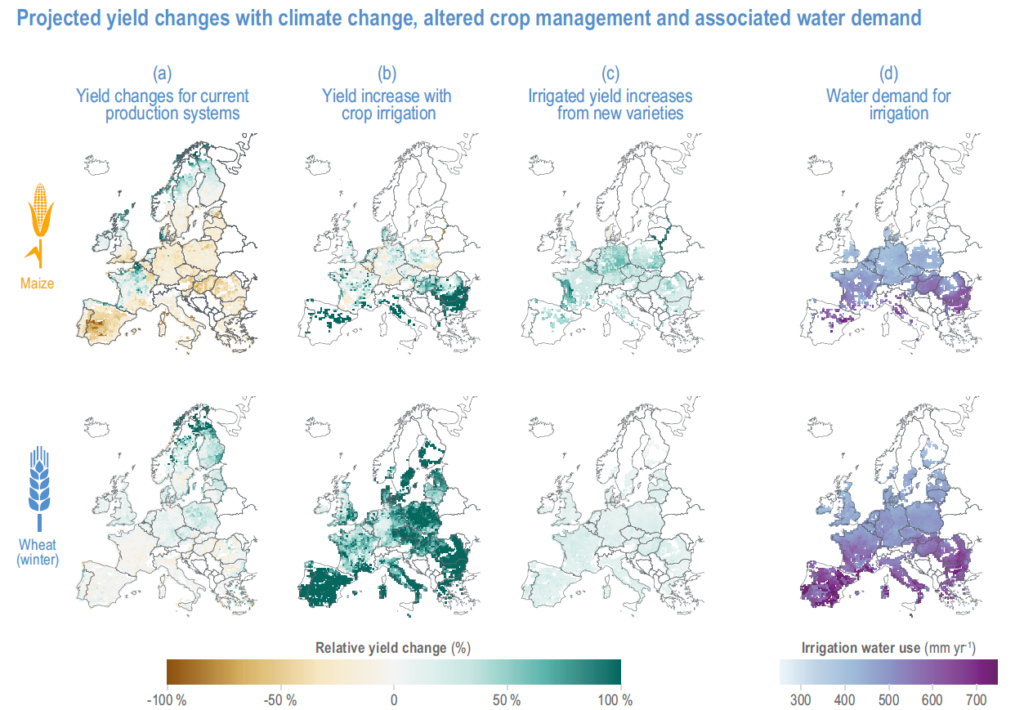
Figure 15. Evolution of wheat and corn yields in Europe following climate deregulation and associated water demand. Source: IPCC 2022. Since AR5, there has been increasing evidence of growing regional differences in projected climate risks. While there is broad consensus on the direction of change, absolute yield losses are uncertain due to differences in model parameterization and representation of adaptation options. Europe’s agroclimatic zones are expected to shift northwards by 25 to 135 km per decade.
Cereals have very different water requirements, depending on their stage of development. The flowering period is all the more decisive as plants are sensitive to water shortage, and the probability of water reserve depletion is high. In the event of poor water supply during this stage, fertilization is poorly ensured, limiting the number of grains per ear. The post-flowering phase up to physiological maturity requires a good water supply to ensure grain filling on the one hand, and to limit scalding on the other, thanks to the cooling capacity of water.
Not all crops are equally sensitive to chronic water shortage, in the sense that some are adapted to very water-limited environments, or that their demand for water does not coincide with the times when water may be in short supply. Crop tolerance to drought depends in part on the depth of root systems. Deep-rooted crops, such as grapes, alfalfa and sorghum, are able to draw moisture deeper from the soil than shallow-rooted crops (e.g. corn and peas), and therefore fare better during periods of water stress. Crops planted in autumn (cereals, oilseeds and protein crops) complete a large part of their cycle outside drought periods. In addition, these plants have the whole winter to sink their roots deep into the soil, enabling them to withstand the dry conditions. Spring crops cycle over 5 to 7 months, growing roots and leaves in drier, warmer periods. They are therefore more exposed to the risk of drought.
While winter crops can continue to be grown under rain-fed conditions (i.e. without irrigation) in the face of climate change (albeit taking into account the difficulties caused by excess water and root anoxia linked to increased winter rainfall), in many situations, particularly in the south of France, many of today’s summer crops will find it difficult to continue growing under rain-fed conditions with a similar yield without irrigation and/or major changes in cultivation techniques. Some spring cereals have become dependent on irrigation, with productivity gains thanks to additional water inputs.
Crops also vary in terms of when water demand is highest. Maize, for example, is concentrated in the summer months, when water stress is at its highest. Rapeseed, winter wheat and winter barley, on the other hand, focus on the autumn and winter months, when more water is available.
At plant level, we can expect phenological cycles to be completely disrupted. Global cycles will be shifted, and certain phases of the cycle will be shortened and/or brought forward, which will necessarily have repercussions on irrigation schedules and therefore on intra-annual dynamics of water withdrawal: irrigation start date, increased needs at the beginning of the cycle, evolution of maximum irrigation needs, decrease in irrigation needs at the end of the cycle. The shortening of the growth cycle could also lead to a reduction in the amount of water used during the season.
Winter crops are likely to experience an earlier crop cycle, with a slight reduction in the duration of key phenological phases. Spring crops should also see an earlier cycle, coupled with a significant reduction in the duration of the grain-filling phase.
Some examples of agro-climatic indicators to monitor:
- Examples of general indicators: precipitation in mm/year, potential evapotranspiration during the spring/summer period, annual water deficit (mm/year), mean annual temperature (°C), sum of annual temperatures based on 0°C, number of hot days (T > 25°C), mean summer temperature, indicators of late or late-night frosts
- Examples of indicators for summer crops: number of days above 32°C, water deficit over the May-August period, number of drought sequences of 10 consecutive days over the May-August period.
- Examples of cereal indicators: number of scalding days (T > 25°C) for the period u May 15 to July 15, water deficit for the period May to June, number of sequences of 10 consecutive scalding days for the period May to June.
- Examples of vineyard indicators: Huglin heliothermal index, September night freshness index, April-September water deficit.
What impact can we expect for animals?
The consequences of climate change on water availability obviously also affect livestock farming conditions. Animals’ physiological needs are directly affected. A cow, for example, has a thermal comfort zone of between 3 and 15°C. Below this, the cow can adapt as long as humidity and air speed are not too low. Above 30-35°C, adaptation is particularly complicated.
Heat stress caused by severe heatwave conditions has a direct impact on production performance. Milk production drops, milk fat and protein levels fall, mastitis develops, animal reproduction is complicated, mortality among young animals increases, and metabolic and animal health problems develop. This state of heat stress is felt not only outdoors, but also in livestock buildings, which are not necessarily sufficiently insulated and where air circulation could be improved. Water shortages could also threaten livestock watering (which accounts for less than 10% of agricultural water use).
Indirectly, and linked to crop production, there are also feed problems for herbivorous livestock due to low productivity of drought-affected grasslands or forage crops (coupled with a change in forage composition and quality). We could also see a combination of excess spring water and summer drought in the same year, adding to the penalties on grassland production penalties on silage maize production. Longer periods of drought and thunderstorms could lead to the abandonment of remote alpine pastures, reducing cultural and landscape ecosystem services and leading to the disappearance of traditional agricultural practices.
Current changes in the availability of grassland for grazing (more grass earlier in spring and later in autumn, and less and less in summer) are prompting all herbivore sectors to consider the type of buildings needed for the summer, as well as forage management on livestock farms. Changes in the seasonality of grassland production are also raising questions about the specifications of various quality labels, such as PDO and others.
Some examples of agro-climatic indicators to monitor:
- Examples of forage indicators: grass growth restart date, grass setting date, early forage harvest date (silage, wraps), first hay date, late hay date.
- Examples of animal indicators: Humidity temperature index for cattle (ITH) = number of days with moderate heat stress (translates into a deterioration in cattle physiological comfort).
Water – an indispensable ally for the agricultural sector
Irrigated and rain-fed agriculture in France
A large proportion of the utilized agricultural area (UAA) is occupied by so-called rain-fed crops, which do not require irrigation. In 2020, in France, only 6.8% of agricultural land was irrigated, i.e. over 1.8 million hectares (in 2010, the figure was 5.8%) – and this was fairly unevenly distributed across the country (Agreste, 2020). Irrigated surfaces exceed 20% in areas such as the Rhône Valley, south-west France and the south-west Paris basin (Fig. 16). And even within these regions, which are relatively well endowed with irrigation facilities, irrigation disparities are considerable, depending on the crop, irrigation method and hydraulic equipment on the farm. In 2020, 34% of corn acreage will be irrigated (versus 40% in 2010), nearly 40% of potato and soybean acreage (versus 51% for soybeans in 2010), half of orchard acreage and over 60% of vegetable acreage.
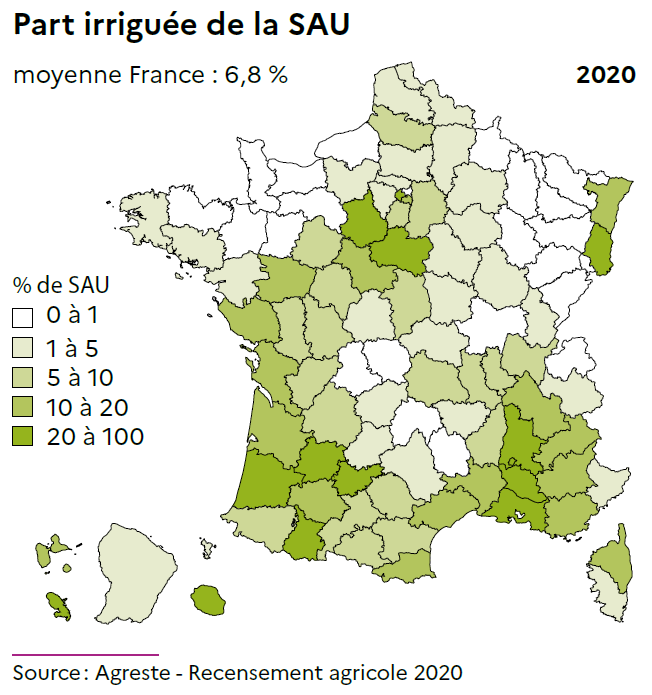
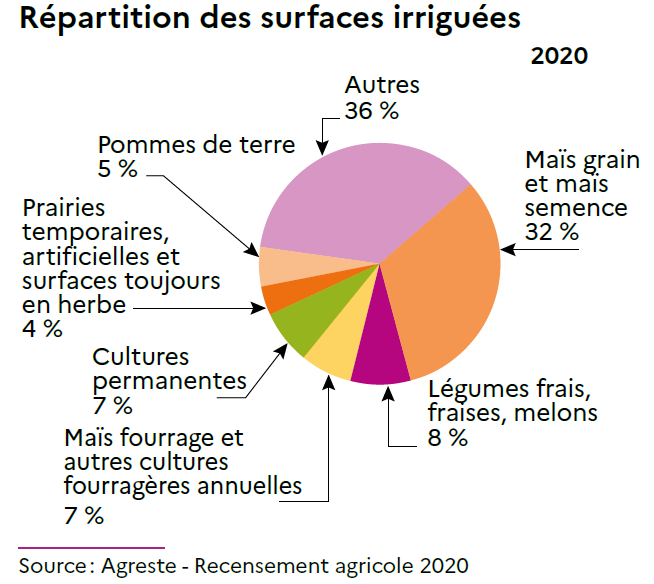
Figure 16. Share and distribution of irrigated land in France. Source: Agreste (2020). In 2010, 15% of French farms were irrigated.
Irrigation management in France is based on two main models: collective management of irrigation perimeters, and individual management by direct withdrawal subject to authorization. In 2010, two out of three farms relied exclusively on an individual water access network. This number has been rising steadily since 1970, while access via a collective network has fallen sharply since 2009 (Loubier et al., 2013). This trend is also observed in the Mediterranean region, where most irrigation is traditionally carried out using collective systems. Farmers connected to a collective irrigation system are thus increasingly disconnecting to use individual boreholes or pumping. The reduction in this form of collective irrigation leads to the weakening of collective structures, since the fixed costs of irrigation investments will be related to a smaller surface area. The financial sustainability of these structures will sooner or later be threatened as more and more members withdraw.
This significant decline in collectively-irrigated areas is due to the combined effect of :
- changes in practices: abandonment of gravity-fed systems, switch to drip irrigation,
- urban sprawl in irrigated areas,
- conversion to dry cereals at the expense of irrigated corn (in the south-west) under the influence of world price variations and changes to the CAP,
- increasingly frequent restrictions on irrigation authorizations in favor of other uses,
- the significant cost differential in favor of individual homes.
Statistical analyses of agricultural census and plot register data can be used to construct a typology of irrigated farms. It is essential to have a reliable assessment of agricultural water withdrawals, over at least one recent year, to validate the modeling of irrigated farms. A typology of non-irrigated farms may also be useful for understanding the impact of irrigation, the relative importance of irrigated production for the different sectors, and possible alternatives in the event of reductions in withdrawable volumes. A dynamic representation of the territory is needed to take into account the effect of inter-annual climate variations (which have a major effect on irrigation) and to assess the impacts of different water availability scenarios and farm adaptations. To do this, we need to model both crop water demand and yield on typical farms and their technical-economic functioning (Serra-Wittling and Ruelle, 2022).
Worldwide, only 20% of land is irrigated. Nevertheless, this land is used to produce 40% of the world’s food in volume. While in France, as we have seen, the irrigated agricultural area is around 7%, in the Netherlands and Greece it is more like 30%, and in Portugal and Spain 15%. Irrigation remains a highly strategic issue, because high value-added crop production generates employment and activity in the region (and this is often what is irrigated). This is notably the case for all market garden crops, horticulture and nurseries, for most seeds and seedlings (contracts are conditional on water control), for field vegetables destined for industry, for half of orchards where irrigation is also used for frost protection, and for field crops when climatic conditions or soil water retention capacity are unfavorable.
It is traditionally estimated that around 90% of the water consumed by agriculture is used for irrigation. The remaining 10% is used for watering livestock and cleaning installations. Irrigation accounts for around 10% of the volume of water withdrawn year-round, but over 50% of the volume consumed in summer, with major geographical disparities and inter-annual variability (see section on Water Withdrawn or Water Consumed?). This demand for water, concentrated over a few months, generally coincides with low-water periods in rivers. Certain crops, including maize, are often singled out for their water consumption, but the subject is generally approached in an over-simplistic way. Corn is a “C4” plant, which photosynthesizes very efficiently and loses less water than other plants (see the section of the dossier on the plant approach). The main issue remains that corn’s demand for water occurs when the pressure on this resource is at its greatest: during the summer. Whether for grain corn (50% of corn surface), feed corn (47% of corn surface), or seed corn (3% of corn surface), irrigating corn enables yields to be several tens of percent higher than similar non-irrigated corn.
Still on the subject of corn, we should bear in mind that irrigated corn acreage fell by 20% between 1988 and 2010. This drop in maize irrigation can be attributed in particular to a reduction in the area of maize grown, especially in regions where irrigation rates were high (Provence-Alpes-Côte d’Azur, Midi Pyrénées…). More generally, the drop in irrigation for certain crops can be explained by the decoupling of production subsidies, and in particular subsidies for irrigated crops, which were a major incentive to increase acreage, by administrative measures to restrict use and reduce overall abstraction authorizations, or by the volatility of agricultural prices and the sharp rise in the price of certain crops compared to others – with the uncertainty of water availability and the most favorable price ratio playing a role in crop rotation choices.
An important question is where this water comes from. Of the estimated 50 billion m3 of water absorbed by agriculture in the European Union, 37% comes from direct abstraction from rivers and streams, 36% from groundwater pumping and 27% from reservoirs, which cover both natural formations (lakes, ponds, etc.) and artificial ones (dam reservoirs, hill reservoirs, etc.), often with multifunctional uses. The recharge of artificial reservoirs is considered as a forced and deferred withdrawal of groundwater or surface water during the water cycle.
The farmer has to juggle numerous parameters to define the quantity of water to be applied, the optimum date and duration of irrigation: availability of water resources, production objectives, crop water requirements, available manpower, period of possible restrictions on use, pedoclimatic context of his plot (soil type, depth, useful reserve…). And these are just some of the practical questions he’ll have to answer: When to start irrigating? How often should you irrigate in the absence of rain, depending on the stage of the crop, so as not to penalize production? How much water should be applied to avoid saturating the soil? When to restart irrigation after a rain? When to stop irrigation to make the best use of the remaining water in the soil without penalizing production?
The irrigation start-up date should not be chosen at random, and is highly dependent on the vegetative stage of the crop produced. Starting too early will limit the plant’s ability to root deeply, while starting too late will deplete the soil’s water reserves before the start of the season. In the same way, to make the best use of the water supplied, the irrigation cut-off date must be chosen so that the water contained in the soil’s water reservoir, which can easily be used by the plant, is exhausted.
Irrigation water will only be put to good use if it is triggered when there is a water deficit in the soil and the crop is at a vegetative stage that is sensitive to drought. In particular, there are two periods of sensitivity to water shortage: a period with an average impact on yield (sensitive period) and a period with a high impact on yield (critical period).
Irrigation must not be allowed to limit water’s natural reserve capacity. The plant must not simply consume water, but use it as efficiently as possible.
Because it can be used to maximize yields, irrigation in France is often associated with intensive agriculture, particularly in the case of corn growing. But irrigation is not a bad thing in itself, especially as in some areas it is simply impossible to produce without water. Researchers have also identified agroecological practices in irrigated systems in certain regions of the southern Mediterranean. We might add that irrigation can be a lever for crop diversification, which is one of the pillars of agroecology.
Let me remind you once again that in France, only 6.8% of land is irrigated, meaning that the majority of land is not irrigated. Clearly, resource management is not just a matter for irrigators. Whether they are irrigators or not, the aim of farmers is to ensure that water penetrates the soil correctly enough to provide a useful reserve. Irrigators must make every effort to avoid falling into the trap of water-dependent, highly irrigated agriculture. In any case, non-irrigators don’t have this leverage. Generally speaking, irrigation in France is not essential to agricultural production, as it may be in arid countries. Instead, irrigation is used to secure yields against climatic risks such as drought (this is also the case for contract crops), limit water pumping into the aquifers, increase average yields, and improve product quality (the development of mycotoxins produced by certain fungi and molds being greatly accelerated by water stress). Nevertheless, we are seeing a growing demand for irrigation in previously non-irrigated areas (e.g. winegrowing in the Hérault region).
In its “Transition 2050” scenarios, ADEME (French Environment and Energy Management Agency) has also carried out projections of irrigation demand for its S1 to S4 scenarios (S1: Génération Frugale. S2: Territorial cooperation. S3: Green technologies. S4: Pari réparateur). ADEME’s work points to a major divergence between the S1 and S2 scenarios on the one hand, and S3 and S4 on the other, with regard to water management (Figure 17). Scenarios S1 and S2 tend towards better control of water consumption (limiting demand) by activating all climate resilience factors. Scenarios S3 and, above all, S4 consume much more irrigation water (via an increase in water supply) in a context of tension over water resources. If we take a systemic approach to all the components of cropping systems, only S1 results in a reduction in water consumption compared to the current situation. S2 maintains consumption at a level comparable to the present. Scenarios S3 and S4 aim to compensate for water shortages with new, non-conventional resources. Scenario S4 moves towards liberalization of the water market, with the possibility of a futures market in water: the economic, environmental and social consequences are as yet difficult to grasp in their entirety, and potentially risky.
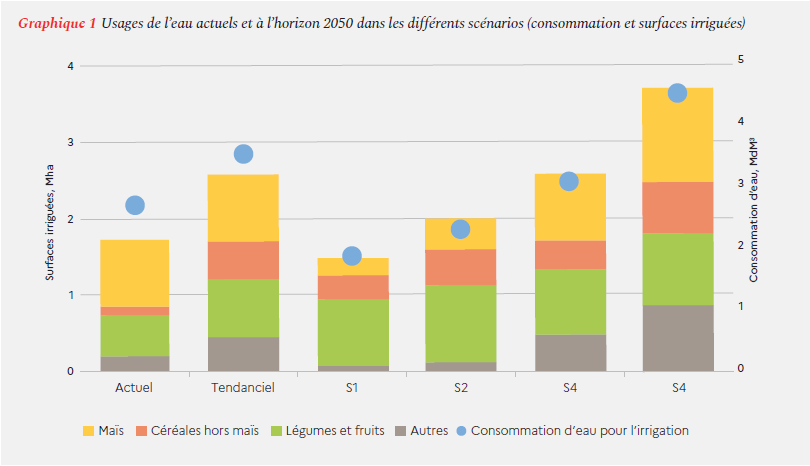
Figure 17. Water use today and in 2050 in the different scenarios. S1: Generation Frugale. S2: Territorial cooperation. S3: Green technologies. S4: Pari réparateur. Source: ADEME Transition 2050
The notion of water productivity or efficiency in agriculture
Water use efficiency is a concept that goes back a long way. Researchers had indeed shown a relationship between plant productivity (the amount of carbon assimilated in the form of biomass or grain produced) and water use. However, water productivity does not increase linearly with grain yield. Over time, water use efficiency, in the common sense of the term, would have increased because grain yields would also have risen for a relatively constant amount of water used. One of the main questions is how plants will react to a changing climate, with changes in temperature, precipitation and carbon dioxide (CO2) affecting their water use efficiency. We’ll touch on this in the section of the dossier on plant ecophysiology, and in particular on the strong interactions between the water, carbon and nitrogen cycles (see the section “Understanding water flows in the plant”).
Crop water productivity, also known as water use efficiency, can be defined as the relationship between crop yield and the amount of water used to produce that crop. It is expressed mathematically as the amount of yield (kg/hectare) per unit amount of water (mm) consumed (i.e. crop evapotranspiration). It no longer only concerns irrigation water, but also the transformation and production process, which includes the plant and its physiology. Water productivity is determined by crop type, physiology and genetics, nutrient availability during the crop cycle and, to a lesser extent, cultivation and irrigation practices. Water use efficiency is easier to measure on annual crops than on perennial crops, where cumulative effects are beginning to appear, and in view of the rooting system, which is difficult to assess, and the diversity of management methods.
In reality, water productivity can be considered from many other angles. For example:
- the technical efficiency of water use, defined as the quantity of agricultural production per unit quantity of water used in production;
- the economic efficiency of water use, which is the value of production per quantity of water consumed, and
- the hydraulic efficiency of water use, defined as the ratio of water consumed by irrigated agriculture to the volume of water supplied.
The main aim of all these proposals is to minimize water input while maximizing crop yields. For example, it’s more profitable to plant maize in France when there’s water, because with water, maize yields are higher. Sorghum, on the other hand, is far more productive than maize when there’s a drought.
Improving water efficiency means producing as much with less water, or more with as much water. Bear in mind that it’s quite possible to be efficient but have a low yield (as is the case with crassulaceous plants) or to be efficient in the sense that you can produce a lot of biomass when the quantity of water allows it, as is the case with corn.
By considering efficiency as a ratio between the quantity of water that actually benefits the crop and the quantity of water applied, the classic definition of water productivity actually makes the assumption that water leaving the plot is akin to a dry loss of water. Other approaches take into account the estimated share of irrigation water potentially available for reuse downstream, i.e. water leaving the plot is considered reusable at the watershed scale.
You can’t simply compare water savings from one year to the next without studying the climatic context of the current years. If, for example, it rained heavily one year, we won’t necessarily see water savings in a drier year. This is why the notion of water-use efficiency should be preferred to that of actual water savings.
Unsurprisingly, water productivity alone gives no indication of the environmental impact and sustainability of economic activities. One solution to this shortcoming could be to assess the intensity of environmental impact in the form of a carbon-coupled balance sheet, for example in terms of tonnes of carbon emissions into water per point of GDP (Gross domestic product). Let’s now turn our attention to irrigation water efficiency (and not just water productivity in general), which is rather useful for assessing the performance of irrigation infrastructures and systems. Irrigation efficiency can be defined as the ratio between the volume of water beneficially used by the crop, i.e. actually transpired by the crop, and the volume entering the plot. By following the path of water from the irrigation network to the plant, we can see that irrigation water efficiency can decrease for a number of reasons (Figure 18). We could even break down this general irrigation efficiency into an application efficiency (closely linked to the irrigation system in place) and a consumption efficiency (linked to the soil-climate context and soil-plant-air interactions). These include :
- Plot input water (Pi), part of which is lost due to leaks in irrigation equipment. These leaks are influenced by the age of the equipment and its poor maintenance. In the case of reels and pivots, these are generally accidental leaks, which are easier to identify. In drip irrigation, leaks caused by damaged sheaths are difficult to identify, even more so when they are buried. Water at the plot entrance can be measured using a meter or flowmeter at the plot entrance bollard. It can also be approximated by knowing the flow rate at the plot entrance and the length of time the valves are open. Billing data from water suppliers can also be used.
- Applied water (Ai), i.e. the water actually leaving the irrigation equipment (nozzle or dripper). In sprinkler irrigation, some of this water may be lost through evaporation due to air temperature, transport (drift) due to wind, interception by the canopy (then possibly evaporation on the canopy). Losses or differences between recommended and applied water can also occur if the equipment is poorly adjusted (wrong type of nozzles, poorly adjusted nozzles, clogged nozzles, use of equipment under poor environmental conditions such as overheating). The irrigation water applied is that which comes directly from the irrigation equipment. It is evaluated by measuring the flow rate of the emitter (nozzle or dripper) multiplied by the irrigation time.
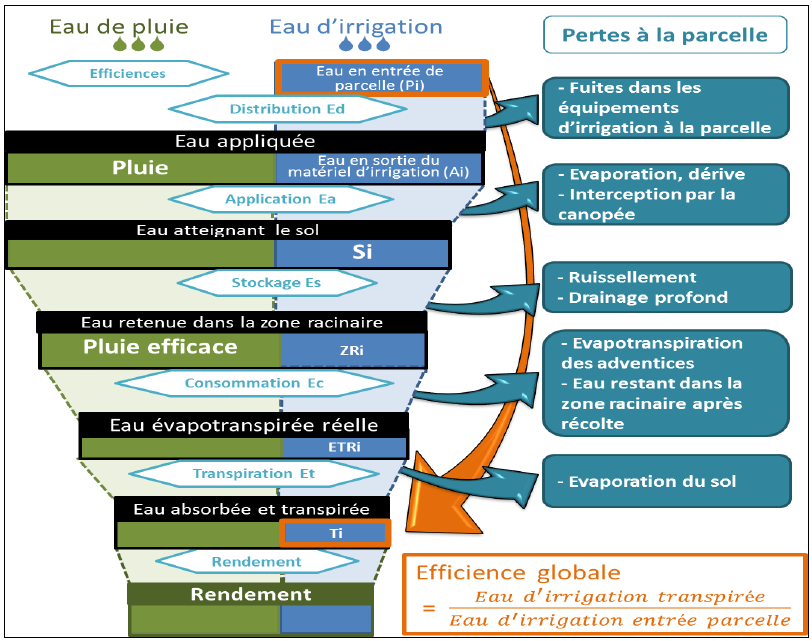
Figure 18. Water efficiency. Source: Serra-Witling and Molle (2017).
- Water reaching the ground (Si), some of which may be lost through runoff or drainage. Rainwater is often measured using rain gauges. For irrigation water, in the case of sprinkler systems, the water reaching the ground can be measured using ground collectors, which also make it possible to assess distribution uniformity.
- Water retained in the root zone (ZRi). Residual irrigation water in the root zone after harvest is lost to the crop over the growing season. It could possibly be reused if a subsequent crop were planted immediately afterwards. Some of this water is also lost through weed evapotranspiration (weeds can also act as a buffer, limiting soil evaporation).
- At this level, rainwater and irrigation water are indistinguishable. Water in the soil can be estimated by sampling and measuring moisture by weight (desiccation), or by field instrumentation measuring moisture by volume (neutron probes, capacitive probes, FDR, TDR…) or voltage (tensiometers).
- Nuclear and isotopic approaches could nevertheless be used to trace the water circulating in the plant by studying the oxygen and hydrogen isotopes in the water. Irrigation water is often enriched in heavy isotopes, making it possible to discriminate whether the water circulating in the plant is irrigation water or rainwater or other water. Similarly, the combined isotopic signatures of nitrogen and oxygen in nitrates can be used to identify and separate the sources of nitrate pollution in agricultural basins.
- Water actually evapotranspired by the crop (ETRi), part of which is lost in the form of water evaporated by the soil. It can be assessed using lysimeter boxes, which measure the amount of water lost to the atmosphere through transpiration and evaporation.
- Water absorbed and effectively transpired by the crop (Ti). It enables the assimilation of carbon dioxide for photosynthesis, and thus contributes to biomass production and yield. It can be measured by sap flow sensors positioned on the plant.
The need to consider water at different spatial scales
Studying water is particularly complicated because we can work on many different scales:
From the farmer’s point of view, irrigation is an agricultural operation. It can be considered from a tactical point of view over the course of the season (what to do depending on what’s going to happen in the coming week?), or from a strategic point of view by planning for the long term (what to do with a water quota or a water reserve?).
From the point of view of the spatial footprint considered, the scales are extremely varied. A Cevenol episode in South France may concern a few square kilometers of footprint, while a drought may cover a very large area. Plant, Plot, Farm, Irrigated system, Management territory, or even Sector are all levels of abstraction that we may need to consider, with different issues each time. At plot or plant level, the problem is often considered from the point of view of productivity and water efficiency. Reflections on water sobriety and adaptation are more likely to take place at the territorial level, because it is at this level that profound changes can take place (development of new rain-fed crop-based industries, implementation of large-scale agro-ecological infrastructures, management of interactions between agricultural and other water uses, etc.).
These are also phenomena that can be studied on different time scales, whether at specific times when water stress is most critical (either at key phenological stages of the plant, or at times of the year when water is structurally in short supply), or on broad time scales to study the trends at work.
Water savings in agriculture excluding digital technologies
Before tackling the section on digital tools dedicated to water management in agriculture, I wanted to emphasize the range of solutions in place to work on the water issue. Whether the proposals are agronomic (changes in farming models and practices), material (overhaul of irrigation equipment and methods) or technological (reuse of treated water, desalination or agri-voltaism), the arsenal is gigantic and much broader than digital technologies themselves. We won’t go into the same level of detail for all these approaches. Generally speaking, we’re talking about strengthening water resources.
A necessary change in the agricultural model
Agricultural practices have considerable scope for limiting water use in agriculture and/or maximizing water efficiency. It is also through the term “nature-based solutions” – within agricultural or environmental practices – that transformations in the agricultural model are generally presented.
It’s already the crop calendar that can be reviewed, for example by modulating sowing dates and densities. These are, in effect, avoidance strategies aimed at shifting the phenological stages of the plant most sensitive to water deficit (often flowering) to a period when water resources are more available, for example by planting early varieties or favoring crops during wet periods. These are also known as avoidance or rationing strategies, and aim to reduce water consumption by, for example, reducing sowing density.
Revising row spacing (inter- and intra-row) will affect the distribution of water evaporation from the soil, transpiration from the canopy, and competition between neighbouring plants. Narrow rows could in fact reduce the time during which the soil is not covered and, in theory, increase water use efficiency if inter-plant competition is not too strong (we can imagine plants with varied root systems).
The diversity and lengthening of rotations could also be interesting levers, even if relatively few studies have demonstrated the precise impact of rotations on water savings. The BAG’AGES project, coordinated by INRAE, has begun to tackle the subject of irrigation optimization and the diversification of mono-specific crops and monocultures.
Even more structurally, switching from water-intensive crops to crops that require relatively little water (and are drought-tolerant, such as sunflower or sorghum), by choosing relevant and adapted varieties, is an obvious option for reducing irrigation water requirements. In fact, it’s not so much the quantity of water per se, but rather the time at which these new crops need it, that appears to be decisive. In regions where water stress is high, and to adapt to the new distribution of agro-climatic zones, it may be necessary to go further and switch from irrigated to rain-fed agriculture (the fact of no longer having access to irrigation removes some room for manoeuvre). Bear in mind that these profound changes in cropping itineraries depend not only on the farm, but also on the market (available channels, product purchase prices, associated agricultural insurance, etc.). Nevertheless, it is these water-saving methods that will ensure the viability of farms.
Soil conservation agriculture (SCA) is also often put forward as a set of agricultural practices that contribute to improving water resources. In particular, SCA helps to improve the water and carbon cycles (better retention of organic carbon) by enhancing soil infiltration properties (microporosity), thereby effectively combating runoff and surface erosion. The organic matter present in the soil helps to structure and consolidate soil microporosity, thereby improving soil water retention (see “Understanding soil water flows” section). Soil conservation practices also improve biodiversity by limiting or severely restricting tillage, particularly in terms of the number of root mycorrhizae, thus promoting the exploitation of soil resources, notably nitrogen and water. Overall, this improves the soil’s overall water stock. The FAO indicates that this organic matter can retain around 20 times its weight in water, and that this is useful not only in periods of drought when moisture is essential for plant growth, but also in periods of heavy rainfall, limiting flooding and landslides, and slowing the flow of water into streams. However, on a regional scale, it is difficult to know whether conservation farming systems save water, as some farmers choose to irrigate their cover crops with the water saved on other cash crops.
Improved water use at canopy level can be achieved by adopting practices that reduce the evaporative component of soil water and divert more water to transpiration. Cover crops are also presented as a way of reducing soil water evaporation. However, these cover crops consume part of the soil’s water, which can penalize the following crop, groundwater recharge and runoff to watercourses. In the particular case of superficial soils, or if rainfall is scarce, cover crops should be destroyed early enough to replenish the soil’s water reserves before the next crop emerges. In addition to cover crops, weeds can also act as soil water buffers.
The rows of hedges and trees present in an agroforestry system have a parasol effect and a windbreak effect that reduce thermal amplitudes during the day and reduce plant evapotranspiration. Although the trees’ root systems draw water from deeper horizons and facilitate the capillary rise of groundwater, competition between the trees and associated crops can be detrimental in dry conditions and on superficial soils, creating local areas of dryness. Green harvesting (topping, trimming, leaf thinning, etc.), particularly for perennial crops, is also a way of juggling future weather conditions and limiting water requirements.
Agronomist Gerard Ducerf also talks about the impact of agricultural practices on water. This agronomist, an expert in bio-indicator plants, is said to have observed, on the same plots of land, bio-indicator weeds of excess water and bio-indicator plants of desertification at thirty centimetres, which would testify to a flagrant lack of water regulator in our plots. Gérard Ducerf also explores a phenomenon he describes as induced hydromorphism caused by agricultural practices. In very dry, very hot periods, the classic tendency is to work the soil when it’s wet. We wait for it to rain before ploughing, sowing and working the soil. However, every time wet soil is worked, hydromorphism sets in. Soil worked in wet weather bursts, the water sticks, drives out the air and the water rises, leaving concrete.
As far as livestock farming is concerned, the salvation for grassland management during periods of drought will surely come from a reduction in stocking rates (number of animals per hectare) during periods of maximum water stress, so as not to bludgeon the grasslands and make it easier for them to recover when the rains return. The selection of more drought-tolerant herbaceous species is one of the techniques to be implemented. Other grazing management practices (winter grazing, dynamic grazing, etc.) will also be of interest.
More indirectly, all practices that encourage water infiltration into the soil and reduce erosion are relevant. Ecological infrastructures in general (wetlands, hedges, grass strips, ditches, riparian forests, buffer ponds) play a fundamental role in reducing runoff and encouraging water storage in landscapes. These infrastructures must be mobilized to slow down the water cycle so as to limit the impact of water surpluses and deficits, optimize groundwater replenishment, develop water storage in deep soil zones and limit soil erosion. Preserving and restoring wetlands, revegetating towns and eroded gully beds, desilting, creating flood expansion zones and re-rectangulating a river to slow it down are all practices to be considered. Implementing these actions, however, is not all that straightforward. While the idea is appealing, in practice, scientific knowledge is lacking and their implementation requires local adaptations negotiated with all stakeholders, as well as experiments that cannot be reproduced, given the specific characteristics of each territory.
Revising irrigation: resilience irrigation and slightly deficit irrigation
In view of the challenges facing water resources, irrigation concepts are also beginning to evolve, and terminologies such as resilience irrigation and deficit irrigation are becoming increasingly popular. These forms of irrigation can be seen as safety irrigation, as opposed to more conventional irrigation, which aims to maximize yields and leads to high water consumption. In a way, we’re moving from maximizing production to inter-annual optimization of farm income under water constraints. Resilience irrigation is sometimes elevated to a concept, a slogan, a kind of irrigation philosophy for maximum resilience of our existing systems.
Deficit irrigation is a first level of change, which involves applying reasoned water restrictions outside critical phenological stages, when yield components are less sensitive to water stress. This approach assumes that substantial water savings outweigh any reduction in crop yield. And these water savings could translate into optimized revenues, due both to lower water costs and to lower energy costs, which have soared in the wake of the war in Ukraine (energy is needed to pump water – more on this later).
Switching to resilient irrigation really is a paradigm shift. We have to accept that, when necessary, we can’t completely satisfy the crop’s water needs, and maintain a properly controlled irrigation deficit. Resilience irrigation cannot be achieved all things being equal. It must be accompanied by changes in crop rotation, varieties and cultivation practices, to ensure that the reduced water input is particularly efficient. However, this is not a new concept for all crops, as it has already been implemented to some extent in viticulture and arboriculture.
Resilience irrigation could be shaped in different ways, for example :
- Technically-controlled irrigation, aimed at delivering the right quantity at the right time in the right place. This optimization requires the combined deployment of tactical decision-making tools (water balance models, etc.) and strategic tools (crop rotation optimization). It also calls for advice and irrigation management, with the installation of sensors and probes, adapted irrigation equipment, communicating meters and high-performance networks. A section of the dossier is dedicated to these subjects.
- Supplementary irrigation (starter irrigation, watering at the right dose at certain critical phenological stages of the plant) to integrate rainfed, winter or spring crops that consume little water into crop rotation, replacing more heavily irrigated crops.
In conventional irrigation, the aim is generally to keep the wilting point relatively low, allowing a wide range of soil moisture. In resilience irrigation, higher wilting point values are allowed, but without exceeding the permanent wilting point (see section “Understanding soil water flow”). The corresponding moisture range will therefore be more restricted, requiring much more precise irrigation control. If we agree to maintain a moisture level that does not guarantee the crop’s full water requirements, we need to be able to estimate the effect of this incomplete satisfaction of needs on crop yield.
Irrigation frequency also affects the way plants read water in the soil. You could compare it to a sort of junky effect, where when you irrigate too regularly, the plant no longer makes an effort to fetch water or adapt to water deficits. As soon as you stop irrigating, the plant starts to suffer terribly.
Notwithstanding the economic and technical aspects of these forms of irrigation, it will obviously be necessary to ensure that they can be set up and deployed, that they are accepted by irrigators, and that they can be imagined on a wider scale than just the farm. We could distinguish two populations of farmers. The first, with a high technical level, or who are in very tight situations in terms of resources (with limited quotas, for example). For this category of farmers, even if water is not very expensive, the fact of running out or not being able to control it could be particularly worrying. The second category of farmers could include those who use supplemental irrigation, often with reel canals, and who are not necessarily very efficient in terms of water use (poorly-adjusted equipment, irrigation in windy conditions, etc.). These farmers can do irrigated and non-irrigated rotations because they have non-fixed systems. Those with fixed systems will tend to irrigate all the time.
The transition to resilient irrigation for a greater or lesser proportion of the farm – depending on the sector or region – profoundly alters the use and therefore the profitability of the corresponding investments (plot or farm equipment and collective networks, where applicable). Farm equipment will need to be fully or partially mobile, or at least more agile, to adapt to irrigation configurations that vary from plot to plot and year to year, from technically controlled irrigation to supplemental irrigation. This implies an improvement in mobile irrigation equipment, which today is generally less efficient than fixed equipment, and the acquisition of new (mobile) equipment for irrigators who currently only have fixed infrastructures. For collective irrigation water development and distribution networks, the development of resilient irrigation means re-examining their economic profitability in the light of lower quantities of water supplied per hectare, and not systematically every year.
Financial support could be provided to help farmers adopt these new irrigation practices. Until now, financial compensation (such as Payments for Environmental Services) has focused mainly on the qualitative aspects of water management, with little emphasis on quantitative aspects. The idea would then be to financially reward the productivity of an irrigation strategy, so as to continue to turn more and more towards deficit or resilience irrigation methods.
A recent CGAAER report analyzing innovative water management techniques in agriculture estimated that 30% of water could be saved in watersheds by combining various irrigation optimization and crop diversification techniques (CGAAER, CGEDD, 2020).
Towards more efficient irrigation equipment
Irrigation in agriculture can take many forms – more or less fine-tuned and more or less technological (Figure 19).
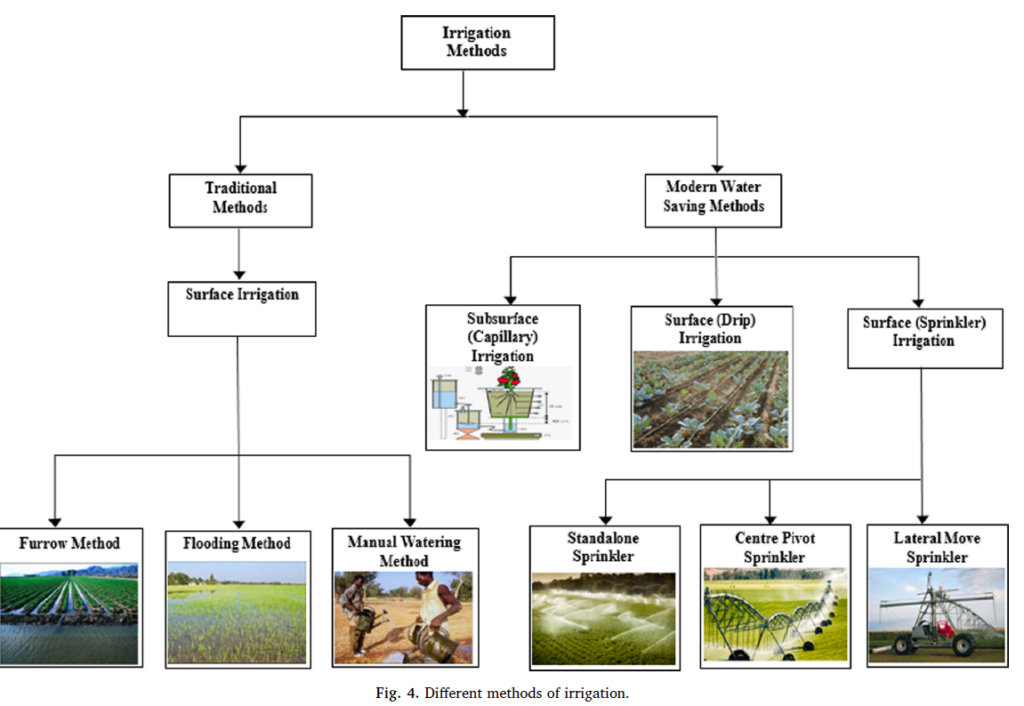
Figure 19. Different irrigation methods. Source: Abiodum Abioye et al (2020).
In 2017, IRSTEA (now INRAE after merging with INRA) published a state-of-the-art report on the efficiency of irrigation equipment for water management. This work also showed the diversity of irrigation methods (Figure 20).
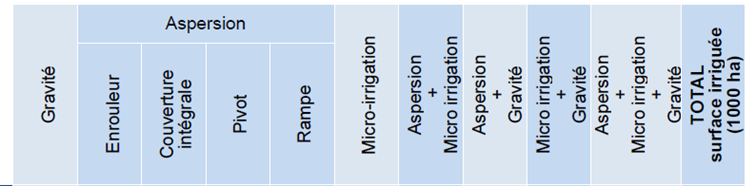
Figure 20: Typology of irrigation equipment. Source: Serra-Wittling and Molle, 2017
In France, the majority of irrigation is by sprinkler systems, at almost 90% (Figure 21). These figures should be seen in the context of the types of crops irrigated in France. The figure refers to irrigability rather than irrigated surface area, because the presence of irrigation infrastructure does not necessarily mean that plots are irrigated.
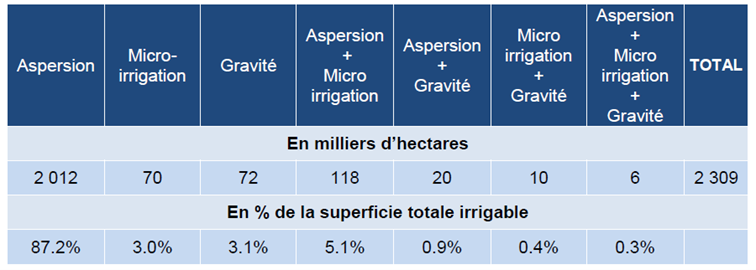
Figure 21. Irrigable areas by irrigation method (in thousands of hectares) in mainland France. Source: Serra-Wittling and Molle (2017) based on 2010 agricultural census data.
The following paragraphs describe some of the characteristics of these irrigation systems.
Drip systems are certainly the equipment with the highest performance capacity (you don’t wet the whole surface, but the soil is wet all the time), but they are still very sensitive to the quality of the water they receive (suspended matter and mineral content in solution). A major risk is therefore the clogging of holes which, if left untended (oxygenated water for biologicals, or acid for chemical problems), will clearly reduce the efficiency of the drip system (and this can drop very quickly). Most drip systems are also used for fertigation. A distinction can be made between surface drip and underground drip.
Surface drip is interesting in that you can see what’s happening (whether it’s irrigating or not) and the system is relatively easy to install in plots. On annual crops, farmers generally use non-reusable systems (disposable sheaths that will be recycled and cleaned at the end of the season).
Buried drip systems are relevant because we water under the plant – the plant then recovers what it needs. These systems are not always buried at regular depths, and are sometimes affected by tillage implements (ploughs and the like). These systems limit soil water evaporation and weed development (since the surface is not watered), and are relatively safe from rodents and other insects that could damage them. On the other hand, these systems are relatively more complex to install, requiring more sensitive maintenance, and it’s difficult to ensure that they’re working properly since they’re not visible.
Cannon reels are not necessarily the ideal agri-equipment for 100% irrigation, and are perhaps preferable for supplemental irrigation. These systems also have a fairly high performance capacity, but are very sensitive to wind. Towed booms equipped with sprinklers are being developed (rather like spray booms) to lower pressures and reduce sensitivity to wind (the equipment is, however, more expensive and bulky, even when foldable). Some have developed guns with adjustable sectors to fit the shape of the plot fairly closely. Others have developed guns whose spray angle can be modified. Winders are also beginning to feature electronic winding regulation – a kind of large spool with a hydraulic motor that draws energy from the water circuit to mechanically wind the tube and maintain a constant speed across the plot (previously, this was achieved by a mechanical device). Little by little, GPS beacons are beginning to be fitted to the cannons to track their movement, with the aim of cross-referencing the position of the cannon with wind data to assess the quantities of water applied to different areas of the plot. Some farmers keep their reels for quite a long time, and convert their old reels into fixed installations, in particular to reduce irrigation on spring or winter crops. The reel is particularly interesting in the context of crop rotation, because it’s a mobile system.
Pivots and front ramps have an economic optimum of around 40-50 hectares at present. These systems can also replace hose reels, which sometimes end up compacting the soil. The use of pivots is becoming attractive, even if it’s for supplemental irrigation, because the second-hand market is developing. These are machines with quite interesting potential for control and various options for reducing intensity, wind sensitivity and pressure.
Another option is to grid the plot with sprinklers, which are aluminum pipes with sprinklers running over the plots. These devices can be adapted to the plot and can operate at very low watering intensities. However, they are also sensitive to wind conditions. The challenge is to produce droplets that are neither too small, which will drift away with the wind or evaporate, nor too heavy, which may clobber the soil (the phenomenon of croute de battance). They are mobile devices because they can be moved from one plot to another. Micro-sprinklers have a shorter range than conventional sprinklers, however, and can be used to work under the foliage of plants above trees (e.g. to treat apple scab, to combat frost, or to cool vegetation during heat waves).
Equipment adjustment, via optimal management and distribution of the volume of water available, is an ongoing area of innovation. Examples include adjusting the angle of the sector watered by a gun (to combat drift losses), adjusting gun speed according to topography (on a slope, the gun puts out less water as it speeds up), winding regulation, inter-passage spacing management, and flow/pressure adjustments.
In 2017, the former IRSTEA also provided some figures on practices linked to the efficiency of irrigation equipment and control (Serra-Wittling and Molle, 2017), including:
- in field crops, savings of 10 to 25% by switching from reel to pivot, and 10 to 35% by switching from sprinkler to drip. Soil probes could generate water savings of 8 to 40%.
- in field market gardening, water savings of 30 to 90% could be expected.
Agricultural irrigation systems only deliver a portion of the water withdrawn to the plant (Figure 18). Modernizing irrigation systems is therefore a potential means of saving water, but with certain limitations. Firstly, part of the water withdrawn for irrigation that does not reach the plant is not lost. It can be returned to the environment by recharging the water table, thus contributing to the proper hydrological functioning of a watershed. The image of archaism or water wastage behind gravity-fed irrigation systems could in fact be counterbalanced by the advantage of operating without external energy (no pumping) and slowing the rate of water flow to the sea by encouraging infiltration into the aquifers.
In addition to the fact that we lack references for certain types of equipment, we also know very little about actual consumption. Water use efficiencies also vary according to the watering needs of the plot. When water needs are low, farmers generally water for comfort, and therefore don’t necessarily pay attention to how they manage water. In tense situations, efficiencies rise because the systems are set to supply the required needs and not to exceed them. However, even if sprinkler or drip systems are more efficient, there’s no question of throwing out the idea of gravity-fed irrigation. Efficiency should not be considered only at plot level (as we have already discussed). Gravity irrigation has secondary effects on its environment and does not only benefit the crop being irrigated. With drip irrigation, since only a small part of the soil is moistened, plant resilience is much lower than with sprinkler irrigation, where plants have access to water in a larger volume of soil.
Modernizing existing irrigation systems (repairing leaks, covering open channels to reduce evaporation, switching to more efficient irrigation systems, etc.) can lead to more rational use of water. However, it should be stressed that, depending on the context, improving efficiency does not necessarily mean saving water. On the one hand, more efficient equipment may require greater technical skill on the part of the user, which, if not implemented, will not result in the expected water savings. Drip irrigation is a highly efficient system, but if the valves are left open all the time, water consumption on the plot will not necessarily be reduced. For example, to design and manage a surface drip irrigation system, it is necessary to have a good knowledge of water distribution, wetting patterns and the spacing between drippers and emitters, in order to avoid greater water loss due to the evaporation process at the surface of the soil and to water use efficiency. On the other hand, water savings can lead to unexpected rebound effects. This is the case, for example, if crops are intensified, the irrigation perimeter is extended, or higher value-added crops are grown that require more water. What’s more, through a phenomenon known as the hydrological paradox, more efficient irrigation can reduce the return of surface water to rivers, thus diminishing the base flows beneficial to downstream users and sensitive ecosystems.
In France, for most networks (and insofar as these networks are on-demand), there is a good match between the optimum distribution that can be obtained at plot level and the capacity of the networks to provide this optimum. Sometimes, in certain cases, discussions take place between the water supplier and the farmer, depending on the agricultural equipment used, to avoid, for example, spraying when there are thermal winds (contrary to what is perhaps intuitive, it is not the heat, when watering at midday, that causes the most losses, but the wind). Evaporation is obviously an issue, but the problem may lie mainly in the degradation of watering uniformity, which generates over-dosed zones (with deep percolation) and under-dosed zones where plants struggle to grow. One consequence is that agris water even more, amplifying the phenomenon.
Outside France, in some places like Morocco, for example, water access systems don’t keep up with demand, or are subject to long water turns, which means that conversion to drip irrigation doesn’t work, because Moroccan farmers have the capacity to maintain a just-in-time water supply. Disasters start to happen when there is no longer a regular supply of water.
Other approaches to strengthening water resources
A quick overview of other approaches to strengthening water resources :
- Some products, such as hydro-retainers, which are not authorized in France, can be placed in the soil to capture water and prevent it from infiltrating or being lost through evaporation. These products can be considered as water augmentation systems.
- Genetic selection (whether natural or not) through the creation of new varieties, the use of new breeding methods, the importation of plant material from hotter and/or more arid regions, or the mobilization of old varieties are all ways of thinking about reducing the water demand of plants.
- Drainage can be used to limit water stagnation in agricultural plots. If controlled, drainage can help maintain a high water table at certain times of the year. If collected, this water could also be reused as treated wastewater. By rapidly evacuating water downstream, drainage can raise questions about its impact on biodiversity, particularly if it encourages the drying out of certain agricultural or environmental zones.
- Reuse of treated wastewater (REUT): In France, the rate of wastewater reuse is less than 0.6%, compared with an average of 2.4% in Northern Europe, and much higher in Mediterranean countries: 90, 85 and 60%, respectively, for Cyprus, Israel and Malta. The 2019 Assises de l’Eau (Water Conferences) set a target of tripling wastewater reuse in France by 2025, but it’s important to bear in mind that it takes a long time for such projects to emerge. The reuse scenarios are extremely numerous (activated sludge vs. reed filters, membrane systems…) and benefit from a socially acceptable image of water reuse because we all assume that replacing conventional natural resources with reclaimed ones reduces the pressure on water bodies. Not all projects make sense, however, because they may also upset a local balance or use a river’s water table (REUT will take water from one place and discharge it in another). Can all reclaimed water be used in agriculture? It’s not necessarily the best question to ask, and the subject raises regulatory considerations (things are changing quite fast), sanitary issues (in France, you can’t mix irrigation water and REUT water), economic costs and energy costs. In agriculture, water requirements are not homogeneous throughout the season. If we are unable to increase irrigation volumes, projects can only replace what already exists. ReuT, like other approaches to boosting water resources, may lead to the creation of new water uses in certain areas.
- Interbasin water transfers consist of reinforcing the insufficient surface water resources of one basin by transporting to this basin volumes of water taken from another basin where water is abundant. Water transfers can take place over relatively short distances, but are also practiced over longer distances, moving water from one basin to another (Durance canal for Marseille, Aqua Domitia for Montpellier and Narbonne). Water transfers between basins are now the subject of questions such as the long-term economic impact on the donor basin and the rights of future generations.
- Wastewater treatment plants (WWTPs) are currently designed to treat water so as not to impact health. They are not designed to reuse water. Today’s water treatment cannot only target pathogens, but must also act on nitrogen and organic matter levels. Current treatment plants are only regulated for organic matter. In addition to dilution phenomena, the hypothesis is that by lowering organic matter levels, the number of pathogens will also decrease. The Dordogne 2050 prospective study has shown that, of all the rural wastewater treatment plants in small towns, half will have, by 2050, a discharge representing 100% of current river flow. The conclusion being that rivers would run dry…
- Agrivoltaism, when used to protect high-value crops from hot spells, helps limit evapotranspiration and water stress in plants
- It might be tempting to store floodwater as excess water. But these volumes are sometimes gigantic. In the case of the Seine, for example, the 800 million m3 of reservoirs managed by the Seine Grands Lacs structure for flood control and low-flow support could only absorb around 20% of a 100-year flood, which is already a lot. It is therefore difficult to intercept all the excess water during a major flood, even for the calmest of France’s great rivers. Nevertheless, we might ask whether water overflowing a water table can be considered as excess water.
- Artificial recharge (AR) of an aquifer (indirect recharge by infiltration, direct recharge by injection, bank filtration) involves the voluntary and controlled introduction of water into a water table to increase its natural recharge for later use or environmental benefit. The water used for artificial recharge is generally surface water, treated wastewater or desalinated water. In France, most of the water used for artificial recharge comes from rivers, since the use of treated wastewater for artificial recharge is not authorized in France, except by specific prefectoral decree. Artificial groundwater recharge has a number of advantages over surface storage, such as limiting water loss through evaporation, maintaining the availability of land above the storage area for urban or rural use, and water purification capacities of the soil and subsoil. Artificial recharge also poses difficulties, not least the risks associated with water storage and its fate in the subsoil. It also raises questions of governance and social acceptability.
- Water desalination (by reverse osmosis or distillation) is a process that makes it possible to obtain fresh water (for drinking or irrigation purposes, although this is rarer depending on the economic cost of desalination) from brackish or salty water (particularly seawater). In addition to its energy cost, desalination produces highly saline water discharges, known as brine. What’s more, the temperature of the effluent discharged can be up to 3°C higher than that of seawater in the case of certain thermal desalination plants, and many undesirable elements are present in the effluent (chlorine, copper, antiscalants, etc.). Another major problem with seawater catchment systems is the aspiration of living organisms into the water circuits – particularly the effects on phytoplankton and zooplankton. Desalination remains a solution of last resort.
- Dams are used to store and regulate water flows – mainly for human use (irrigation, power generation, flood management…). In France, the 530 large dams already listed store 12 billion m3. That’s almost 6% of all runoff in France. In the event of flooding, dams can be opened to release water which, in excess, will mainly flood agricultural plots or flood plains. Some dams are designed to reduce the maximum river flow during a flood.
- Lastly, we propose the replacement reservoirs and basins, which we’ll discuss at the end of this dossier. The number of reservoirs is estimated at over 600,000. Even if most of them are small, the volumes stored during high-water periods are not negligible, even in relation to runoff. As far as groundwater is concerned, the water table levels chosen for substitution withdrawals are generally well below the overflow level. In the Marais Poitevin, for example, where replacement reservoirs draw on the water table, the levels chosen are closer to the minimum observed in winter than to the overflow level. In certain types of reservoirs, water is nevertheless considered to be in excess, as it would otherwise flow into the sea. However, this overlooks the many chemical, physical, biological and ecological feedbacks. In the Mediterranean basin, for example, which is a quasi-enclosed sea, freshwater inflows have been greatly reduced by the construction of dams that have created reservoirs (and the evaporation of water bodies) and the use of this water for human activities.
These solutions, whatever they may be, must be the subject of sound benefit-risk analyses. It is important to bear in mind that water management efforts do not necessarily translate into water savings. Water efficiency or productivity can be increased at a constant water regime, provided that the output variable (e.g. yield) also increases. Particular attention must also be paid to the rebound effect, or the fact that a gain in efficiency achieved by a technology is quickly offset by an adaptation in behavior, which ultimately leads to greater use of resources. It’s all a question of balancing the benefits against the foreseeable long-term risks.
Some preconceived ideas and misleading language
No, not all droughts are created equal
The number of drought decrees increased in 2022, appearing earlier and earlier in the season and affecting more and more départements. Droughts are the product of several phenomena that can combine (Reghezza and Habets, 2022):
- meteorological drought, resulting from lack of rainfall
- agricultural drought, resulting from a lack of water in the soil;
- hydrological drought, resulting from low groundwater levels, surface reservoirs and rivers.
Note that when there’s no water in the air, it’s called a heatwave – and when there’s no water in the ground, it’s called a drought.
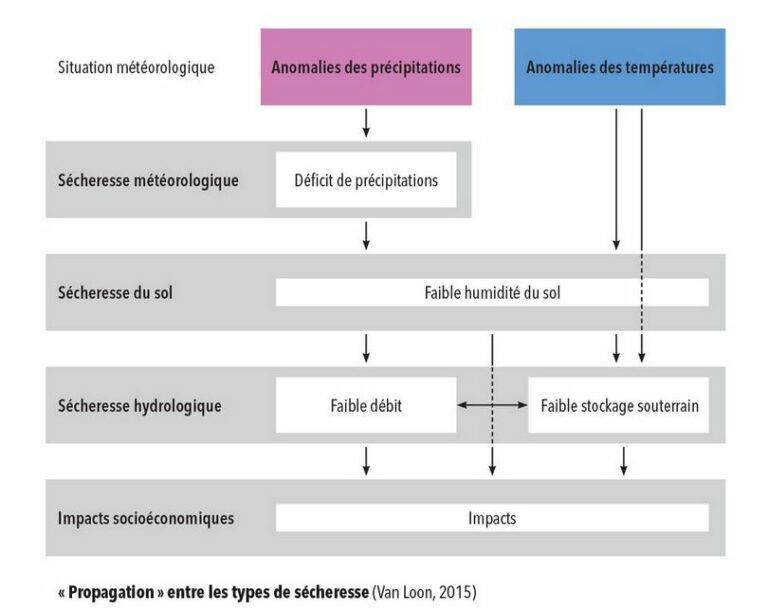
Figure 22. The different states of drought. Source: Van Loon, 2015 – from Reghezza and Habets’ brief on the Bon Pote site (2022).
These differences are important for understanding the impact of rainfall. The winter droughts we are experiencing, and in particular this year of 2023 with its unusually dry and warm months, mean that hydrological recharge is highly inertial. In France, even if recent rains have effectively recharged agricultural soils, water tables remain dry, with records still very low (see BRGM maps on figure 11 in the dossier). The amount of rainfall that is actually effective is lower. Just because rivers are flowing on the surface and soils are finally being recharged doesn’t mean that groundwater drought has abated. This year’s rains came after the end of the recharge season.
The rains we’ve been experiencing have helped to alleviate the dryness of agricultural soils, thus avoiding the need for irrigation. This is all the more interesting news given that the water tables will not be able to support irrigation. The impact of these rains can be immediate (resumption or acceleration of growth for plants, particularly forage, young market garden plants, reduced risk of fire), provided that the rains are not too intense and do not generate runoff.
Water vapour is a greenhouse gas
Water vapour is a greenhouse gas and therefore contributes to climate change. It is not the driving force behind global warming (which remains the emissions of CO2, methane, etc.), but it does contribute to it through an amplifying effect. Generally speaking, water vapour plays a major role in the greenhouse effect, with water vapour accounting for 60 to 80% of the energy captured and returned to the surface by the greenhouse effect.
A warmer atmosphere can contain more water in vapour form, and so an initial warming of the air will lead to an even greater warming due to the increased concentration of water vapour in the atmosphere (water is available in essentially unlimited quantities in the oceans, hence the rapid adjustment).
Nevertheless, the concentration of water vapour in the atmosphere varies only globally as a function of temperature. This is mainly due to the very short residence time of water vapour in the atmosphere (of the order of 10 days), which doesn’t give water time to permanently alter the Earth’s energy balance, and to the fact that water vapour will tend to condense into liquid water (clouds, then precipitation) when its concentration becomes too high. Direct human emissions of water vapour (from power plants, for example) therefore play no role in global climate change. They can, however, be significant at local level, notably via irrigation, which can modify rainfall distribution and energy flows at regional level. As we saw earlier in the dossier, deforestation also has a strong impact on regional climate, by modifying water flows (trees transpire) and therefore rainfall patterns (we have already discussed the different water cycles).
As air warms under the effect of rising GHG concentrations, it contains more water vapour (+7% per additional 1°C). This phenomenon traps even more heat, amplifying global warming. This is what we call a feedback loop: one phenomenon (warming due to CO2 and methane emissions) produces another (increased water vapour concentration in the atmosphere), which increases the first (global warming), and so on.
Climate models take full account of water feedback (after all, it’s a relatively simple mechanism – temperature rises, so does evaporation over the oceans). The uncertainties in climate models lie above all in the clouds, because we still don’t fully understand what will happen to cloud cover. The cold subtropical seas (Canary Islands, California) are often covered by low, very dense clouds, which play a very important role in the earth’s albedo. Nor do climate models take into account (or at least very imperfectly still) the impact of irrigation or deforestation.
Water withdrawal or water consumption?
In France, agriculture, mainly through irrigation, accounts for around 9% of water withdrawals, but 48% of consumption (Figure 23 – figures vary slightly from one source to another). This is the sector that consumes the most water, in the sense that the water withdrawn by plants is not returned locally. This water is evapotranspired and re-enters the cycle in the form of vapour, before falling elsewhere in the form of precipitation. From a local point of view, the water is therefore lost, but in reality it should be said that it is displaced in the cycle.
Irrigation withdrawals can be compensated for by releasing water from upstream dams. Hill reservoirs or other storage infrastructures (such as basins) can also be used (more on this later).
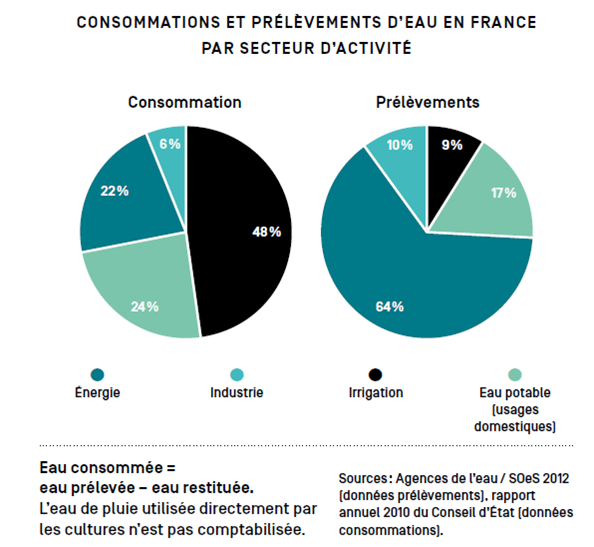
Figure 23. Water consumption (left) and withdrawals (right) in France. Figures vary from one source to another. The government, for example, points out that the energy sector accounts for 50% (instead of 64%) of water withdrawals, and that 98% of the water withdrawn by power plants is returned to the environment. Note that water withdrawals have been falling since the early 2000s except in the agricultural sector (MTES, 2018)
The energy sector is the largest user of water (over 60%), mainly due to the cooling needs of nuclear plant condensers. Each nuclear unit consumes between 50 million m3 and 1.5 billion m3 per year, depending on its power output and, above all, whether cooling is carried out in a closed circuit (with a cooling tower) or in an open circuit (the most water-hungry). The proportion of open- and closed-circuit reactors installed in France is virtually equivalent. These substantial water requirements explain why nuclear power plants are built either on rivers with high flow rates, with upstream hydraulic structures built to regulate flows (reservoirs supplying water in the event of insufficient flow, or conversely storing excess water to prevent flooding), or on the seashore or in estuaries. The lower demand for electricity in summer nevertheless makes this constraint bearable for the power generation system.
Drinking water accounts for 17% of the volume abstracted in France (of which around 30% is lost through leaks in the transport and distribution networks). Bathing and showering are the main uses of drinking water in France (around 40%).
Unlike the water used in agriculture, the water used to cool conventional or nuclear power plants, or the water used for domestic consumption, is partially or totally returned, usually close to the point of abstraction. More specifically for the nuclear sector, when the reactor is cooled in an open circuit, almost all the water is returned to the environment downstream of the cooling system, at a temperature higher than the temperature of the water when it was drawn off (the heating of the water is of the order of 10 to 15 degrees, reduced to a few degrees after mixing with the water drawn off downstream). When the reactor is cooled in a closed circuit, the water withdrawn is only partially returned to the environment, since 20 to 40% of the quantities withdrawn evaporate in the cooling towers. Provided certain criteria are met, water from power plants can be reused, particularly in terms of temperature and quality. Discharge points may nevertheless be located at some distance from the point of abstraction, particularly in the case of diversions (canals).
A quick aside on drinking water. The French drinking water network alone represents more than 900,000 kms. The annual pipe renewal rate is 0.67%, i.e., on average, a refurbishment every 160 years, whereas the lifespan of a pipe is around 80 years. Add to this an average leakage rate estimated at 20% (sometimes as high as 50% in some areas), and you have billions of m3 of water leaving our pipes every year, which is not distributed directly to residents (but which is potentially returned to the environment…).
The distinction between water abstracted and water consumed is, however, rather artificial. Water considered as consumed is sometimes largely returned to the environment, whether in the form of drinking water distributed to the consumer or water used for irrigation, a significant proportion of which may not reach the plant but instead infiltrate the soil. This return to the environment generally takes place in close geographical proximity to where the water was taken, since irrigation networks, like drinking water networks, are local networks.
Low-water flows, biological flows and ecological flows
More generally, although some may find it useful to distinguish between water withdrawn and water consumed, it is above all the impact on ecosystems that needs to be considered. This raises the question of thresholds around the different volumes of water that can be abstracted and consumed. The difficulty lies in the fact that the notion of flow differs according to ecological, technical and economic considerations (Figure 24).
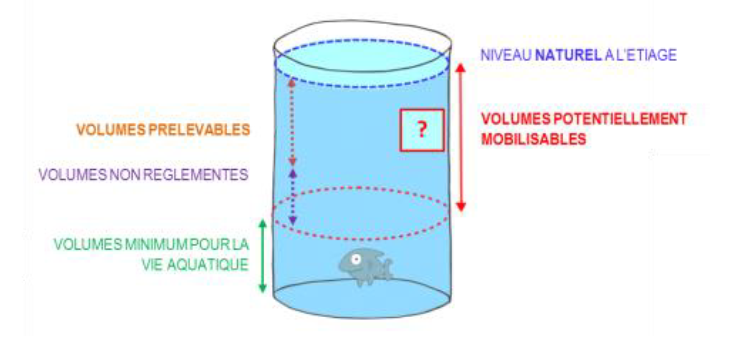
Figure 24. The different notions of withdrawable and non-withdrawable water volumes.
In accordance with the European Water Framework Directive and the 2006 Law on Water and Aquatic Environments (LEMA), (maximum) volumes that can be abstracted are defined in zones with structural water deficits, or water distribution zones (ZRE). These volumes obviously include all the various uses, including irrigation.
The regulatory framework requires the definition of target low-water flows (DOE in french) to satisfy all uses on average eight years out of ten, and to achieve good water status. The value of the target low-water flow is defined by reference to the value of the minimum mean monthly flow at the five-year dry frequency (QMNA5 in french), whose return period is by definition close to the objective of satisfying the balance of needs and resources, i.e. on average the 8 years out of 10 mentioned at the beginning of this paragraph. These target low-water flows are generally obtained following studies of withdrawable volumes (EVP in french), based on detailed hydrological or hydrogeological expertise, which need to be regularly updated.
Compliance with low-water target flows is a major constraint that contributes to aggravating the imbalance between the needs of various uses and summer availability, especially as maintaining a low-water target flow (DOE) already requires ensuring that a certain quantity of water flows through each section of the surface network. Set too high, this flow prohibits all agricultural abstraction at certain critical times of the year. Its very principle raises questions in areas where the total drying-up of a watercourse in summer might be acceptable, rather than seeing the entire agricultural activity of a valley come to a halt. Debates already exist on the evaluation of the available resource, because the models used to establish low-water target flows (DOE) are necessarily based on working hypotheses and extrapolations.
The requirements of these low-water flows theoretically translate into :
- a DOE to meet the ecological flow required to satisfy the needs of the aquatic environment. Where several ecological flows have been defined, the DOE will aim to respect all the ecological flows of the management unit in question,
- a DOE whose maximum value is limited by the natural monthly low-flow rate of the watercourse, excluding any abstraction or artificialisation of the water regime in the catchment area. It is currently considered that, except in special cases, there is no reason to set a better objective than the natural state observed 8 years out of 10 (or 4 years out of 5).
Potentially mobilizable volumes are obtained by calculating the difference between the floor flow of the DOE and what monthly hydrology is able to guarantee 4 years out of 5, i.e. the dry five-year monthly flows of each month. Each DOE range is associated with a monthly Potentially Mobilizable Volume (PMV) range. When monthly DOEs are aggregated into a single seasonal DOE, and the potentially mobilizable volumes are smoothed out over several months, we collectively run the risk of missing the objective of satisfying needs and uses at the heart of the low-water months. Similarly, DOEs and biological flows defined on a monthly basis do not protect against daily droughts.
Ecosystem preservation issues have led to the definition of biological and ecological flows. Biological flow is understood as the flow in the bed of a watercourse that enables the general functioning of aquatic living communities located in the upstream watershed. Ecological flow integrates with biological flow the additional objectives of good water status (physical-chemical, etc.). These two concepts are distinct from the Minimum Biological Flow (MBF), defined as the minimum flow that must be maintained downstream of a structure in the watercourse and that guarantees the life, circulation and reproduction of the species living in the water. The notion of Minimum Biological Flow is an instantaneous value, linked to a structure and to the section of waterway directly downstream. It leads to the definition of daily instream flows to be respected downstream of the structure.
Generally speaking, “biological flow” and “ecological flow” are monthly flows designed to guarantee the proper functioning of aquatic environments, as part of an integrated approach to the structural and balanced management of water resources on a catchment scale. These different flows depend in particular on :
- flow values at a given time, with particular attention to minimum and maximum values,
- frequency with which certain flow values are observed. For floods and low-water periods, we speak of return periods (annual, five-year, ten-year, hundred-year),
- duration of flow above or below a threshold value, predictability of events, regularity with which certain hydrological episodes recur and on which biological strategies are adjusted,
- stability, speed of flow changes over a short period.
The low-water target flow (DOE) was originally designed to ensure equitable sharing of water resources between upstream and downstream sections of a river. The intention was that upstream users should not deprive downstream users of water (for irrigation, for example). Today, instream flow is more in line with environmental concerns, such as managing water reserves for surface and groundwater recharge, guaranteeing the proper circulation of sediments, and preserving biodiversity by ensuring ecological continuity along the entire length of the watercourse, in order to conserve flora and fauna. While maintaining an instream flow may appear to be a virtuous principle for the balanced management of watercourse flows, it can actually lead to favouring upstream over downstream, even though drying up downstream can cause more problems than drying upstream. Moreover, maintaining an instream flow may not be possible when rivers dry up completely during low-water periods. In addition, where pumping and irrigation are important, it is very difficult to know what the normal flow level of a river (natural modulus) is. It may therefore be necessary to carry out calculations to estimate the reconstituted natural modulus (this is what the Explore 2 project is working on, for example).
From a hydro-ecological and fundamental ecology point of view, setting these flow thresholds is particularly complicated because we lack knowledge on the subject. For example, the conditions under which replacement irrigation reservoirs are filled in winter raise the question of the environmental impact of a reduction in de bits or a drop in piezometry during this period (we’ll come back to this in the context of recent events around Saintes Solines).
An alert threshold flow (DSA) and a Crisis Flow (DCR) are thresholds used for crisis management. These thresholds, expressed as average daily flows, are operational thresholds that are compared daily with the daily flow. By comparison, the DOE is a monthly mean value with a probability, intended for retrospective analysis. On fast-drying rivers, the DSA value may be higher than the DOE, in order to allow for different levels in the restriction system and to avoid reaching the DCR.
All flow values must now be questioned in the light of climate change and its impact on hydrology. The impact of climate change on river hydrology will generally lead to a drop in natural river flows. The impact on the environment of this drop in flow will be particularly aggravated during low-water periods by the rise in water temperature. At constant abstraction levels, current DOEs may no longer be respected 8 years out of 10. However, the flow requirements of aquatic environments will not diminish, and may even increase as water temperatures rise.
To work in the most detailed way possible, the description of each use must be as exhaustive as possible: nature (withdrawal and/or discharge), purpose, location, annual and monthly volumes, spatial and temporal distribution of withdrawals, withdrawal flow rates, minimum operating flow, resource concerned, etc. Particular attention must be paid to identifying the groundwater resources mobilized and the interactions between these and surface runoff. All water-dependent uses are concerned, whether direct, diffuse, water-consuming or not. Solid hydrological studies must consider (Agence de l’eau Loire-Bretagne, 2022):
- an analysis of hydromorphological characteristics and their evolution, whether natural or influenced,
- an analysis of flow-quality relationships for watercourses, with an analysis of physicochemistry and hydromorphology,
- a hydrogeological analysis whenever the underground environment is concerned: interannual piezometric trends, groundwater-river interactions, impact of piezometry on surface environments,
- consideration of the status of water bodies in the basin in question,
- taking into account the biological reservoirs identified in the basin in question,
- taking into account the register of protected areas,
- taking into account, for coastal basins, the freshwater needs of marine species, without excluding the definition of salinity targets at relevant nodal points.
Water’s virtual footprint
Although still relatively unknown, the concept of “water footprint” or “virtual water” has little to envy to that of the carbon footprint, now widely democratized (the RSEAU project was recently proposed to integrate the water dimension into corporate accounting). In the end, the underlying idea is quite similar. The water resource is quite specific in that it is one of the only resources where we currently have well-spatialized indicators and monthly time steps for calculating impacts (this is a major advance and added value for making impact assessment operational). For carbon-related issues, spatialized indicators make little sense in the final analysis, as the subject is global in scope. For nitrogen, the localization of emissions is important, but no sufficiently consensual methods yet exist.
Nevertheless, it is important to realize that, in general, the inventory requirements of water footprinting methods differ considerably. The availability of the characterization factors required for an LCA (life cycle analysis) and the quality of the data available are also subject to considerable variability. Due to a lack of data, some databases, such as Ecoinvent, only contain freshwater inflows, but no wastewater outflows, and therefore allow water use to be determined, but not water consumption. Other databases, such as GaBi, provide figures on use and consumption, but only take into account part of the uses and electricity production.
The notion of water footprint is often misunderstood. Several examples can testify to this. Take the example of Levis jeans. The Levis company ran an ad promoting the idea that if everyone stopped washing their jeans (or reduced the number of times they were washed), they would save drinking water. Switching to a machine consumes electricity but, in addition to the water evaporated when the jeans are dried, the water used in the machine returns to the sewage treatment plant and to the rivers (and in a way goes straight back into the environment). So no drinking water is saved. On the other hand, the water used in agriculture to produce the cotton used to make the jeans is largely evapotranspired by the plants and returns to the large water cycle or the small continental cycle, and is therefore potentially lost locally.
The equally well-known figure of 13,000 liters of water used to produce one kilo of beef is based on Life Cycle Assessment (LCA) methodology, which accounts for rainwater as if it were line-cleaning water in a factory, i.e. without taking the water cycle into account. The LCA method, which comes from industry, generally neglects the effect of water recycling, and this is where the problem lies in the fact that the vast majority of water consumed in agriculture is recycled (it goes back into the water cycles), including rainwater in particular. To say that 13,000 liters of water are consumed to produce one kilo of beef is particularly false. The amount actually consumed by our kilo of beef within the great natural water cycle is less than 1%. In France, calculations taking these limitations into account give water use levels of 20 and 50 liters per kilo of beef, which is already significant but has nothing to do with the order of magnitude. The crude LCA methodology is not particularly well suited to work on water consumption in agriculture. We could also add that aggregating blue and green water into a single figure masks the advantages of rain-fed agriculture over irrigated agriculture. It might also be interesting to count water not only in terms of kilograms of produce, but also in terms of kilograms of protein or kilocalories produced.
Like the “climate change” impact category and the carbon footprint (which are ISO-standardized), the consequences of water use can be assessed as an impact category within the LCA framework, or as a stand-alone water footprint. In this section, we will focus on individual water footprints as a particular form of LCA. Methods for analyzing water use in LCA can be divided into accounting and impact assessment approaches. Accounting methods remain at the volumetric level and provide the basis for any subsequent impact assessment.
In contrast to the volumetric approaches of virtual water and water footprint, LCA aims to assess regional impacts in addition to the volumes of water used throughout a product’s life cycle (Berger and Finkbeiner, 2012; Berger et al., 2016). This different interpretation of the water footprint as a volumetric or impact-oriented indicator has sparked fierce controversy within the scientific community. Some researchers stress the need for further interpretation, as 1 m³ of rainwater consumed in Brazil is not comparable to 1 m³ of groundwater consumed in Egypt (the notions of volume used and volume consumed thus have different spatial meanings). Other authors argue, on the contrary, that the appropriation of freshwater on a global scale is more important because impacts are difficult to predict, and water is a global resource that is virtually traded through all products that required water at some point in their manufacture. Some authors add that impact-based water footprints without physical interpretation are completely meaningless, giving rise to questionable weighting choices, especially as the local environmental conditions influencing impacts would be rather poorly described. Nevertheless, the general consensus tends to be in favour of mixed approaches, combining both impact and volumetric methods.
The fact that there are several characterization models available for water consumption should not be seen as a problem in itself at present. On the contrary, it reflects the complexity of reality. As long as there is no general consensus on an impact-based water footprint assessment that deals consistently with all impact pathways, several characterization methods need to be applied in order to analyze the different implications. The United Nations Environment Programme (UNEPà has commissioned an “Aware LCA” indicator to define the most appropriate method for calculating water footprints according to these uses.
The water footprint concept has the advantage of raising awareness of our dependence on water for everyday consumption, but it says nothing about the conditions under which this water was captured. Products with a low water footprint but manufactured in a place or at a time of high water stress, or in a place where the resource is highly sensitive, are more problematic than products with a high water footprint from geographical areas with abundant resources. For example, water consumption for irrigation is four and seven times higher in Italy and Spain respectively than in France. As a result, the virtual water imported for our food (mainly in the fruit and vegetable sector) represents a volume equal to that which France devotes to irrigation. Under the guise of optimizing water use and seeking net water savings at all costs (we only subsidize what we can use less of), we tend to fall into simplistic, mono-factorial approaches that fail to grasp the complexity of the world. A tomato irrigated in the Jordanian desert on a borehole 600m away with desalinated water is much less virtuous from the point of view of the water cycle than the market gardener next door who works with floods. Almonds score very poorly in terms of water use, because Californian almonds are very water-intensive, whereas we’re perfectly capable of producing almonds with a much lower water impact, on soils that are highly resistant to drought.
The spatial and temporal aspects of water consumption are fundamental. The spatial distinction of water consumption is essential because of the great variability of the associated impacts. We could also add that a watershed perspective is necessary, since water flows transferred from one watershed to another must be taken into account in the assessment. Indeed, if withdrawals and discharges take place in different watersheds, the result is consumption in one watershed and negative consumption in the watershed where the water is discharged. In fact, it would be possible to go one step further by adopting the concepts of atmospheric basins theorized recently (see earlier in the dossier), since the masses of water exchanged in small and large water cycles are gigantic.
Temporal aspects are generally not taken into account in existing databases, yet they are fundamental given the extent to which water availability and demand vary from one moment to the next. The environmental effects of water use depend on when it is used. And these temporal issues are particularly important for agricultural production, where there is great variability in water use from one month to the next, depending on the plant’s growing season. One way of dealing with this risk could be to use a stress coefficient based on the number of days during which plants have no access to water during their growing season. It is often overlooked that such a level of temporal detail requires taking into account intermonthly storage capacities, which can buffer periods of water shortage throughout the year. A consumption/availability ratio for a dry month may overestimate the shortage when water reservoirs created in previous wet months are ignored. Alongside this, the temporal resolution of water scarcity assessments also conditions the spatial resolution required of the assessment. Large basins can have flow durations of several months between spring and mouth, making a monthly assessment difficult.
Most methods focus on blue water consumption. Green and grey water are mainly taken into account by stand-alone methods to deal with the evapotranspiration of rainwater from agricultural products and the degrading use of freshwater, respectively. Water consumption from soil moisture (green water) is a controversial issue in the water footprint, as it is a natural water source received through land use. Only the difference between the consumption of this water and the natural situation can be taken into account. Consequently, these indirect forms of water consumption must be linked to land use inventories.
Further research is needed to determine the fate of evaporation in a spatially explicit way. If recycling of atmospheric moisture is taken into account, current water inventories will change radically, as evapotranspired water is no longer considered as consumed per se. There could even be water credits resulting from the precipitation of water vapour created synthetically during the combustion of fossil fuels. In this way, cars can have a positive carbon footprint but a negative water footprint if they are driven in regions where continental evaporation recycling is high.
With regard to green water consumption, for example by agricultural plants, it should be noted that natural vegetation also causes evapotranspiration, which can be even higher than that of agricultural plants. This is why some authors suggest determining the “net green water footprint”, i.e. the difference in evapotranspiration between agricultural land and natural land. However, the relevance of net or total green water consumption is debatable, as soil moisture is only available to local plants and cannot be used by surrounding ecosystems or abstracted for human needs.
Perhaps the real question to be addressed is the extent to which the green water footprint affects the availability of blue water. This is a particularly complex issue in that it is closely linked to land use. When the effects of land use change on blue water availability are considered, care must be taken, as the conversion of natural land into arable land can lead to an increase in blue water availability due to changes in surface runoff and groundwater recharge patterns. A strange or surprising consequence all the same is that one could imagine offsetting one’s footprint on blue water through changes in land use, such as deforestation. This, however, ignores the fact that natural vegetation is an important foundation of the global water cycle, and that changes in land use affect this cycle.
Most existing impact assessment methods do not distinguish between surface water use and groundwater use (groundwater or surface water stocks, which can buffer water shortages over time). This can be important, as an activity may consume surface water but have a negative groundwater consumption. Flood irrigation in agriculture is an example: in general, river water is partially consumed as blue water, but it partly feeds groundwater, resulting in negative consumption (i.e. groundwater production) and can have an overall beneficial effect, even if there is net water consumption.
Some new LCA concepts are also beginning to emerge:
- Territorial LCA, a kind of extended LCA that looks at several specific functions provided by the territory. The main reason put forward for this is the limitation of conventional LCA approaches – centered on a specific function – which struggle to take into account the complexity and diversity of exchanges within a territory (pumps for water abstraction, water supply systems with canals, irrigation infrastructures, water treatment infrastructures). Consider, for example, the reuse of wastewater as part of a circular economy.
- Foresight LCA, which looks ahead in time and assesses how impacts may or may not contribute to climate change.
- Dynamic LCA, which adds temporality to characterization and impact factors by considering that they can evolve over time.
Digital technologies for water management
Most of today’s digital water management tools are integrated into irrigated agriculture. This is obviously a very good thing, given the demands we have to make on this scarce resource, but once again, the amount of irrigated cultivated land remains a minority compared to the amount of non-irrigated cultivated land.
Although you’ll see that the tools are fairly evenly distributed across all the proposed categories, it’s certainly the digital tools as measuring instruments that we hear the most about. These tools are perhaps more concrete and tangible than the services intended to support irrigation management, but the general impression is that water balance tools based on modelling are not really operationalized.
I could be criticized for saying that measurement tools can be used to assess the state of water resources, whether crops are irrigated or not. I agree. Nevertheless, it has to be said that the messages conveyed more regularly revolve around irrigation support than anything else (or perhaps disease risk and development stage forecasts for weather stations).
You may be surprised to learn that, particularly when it comes to measurement tools, approaches to monitoring water resources are extremely varied. Some approaches are more consensual than others, of course, but generally speaking, everyone has their own complaints and tends to feel that their approach is a more accurate reflection of reality than the other.
In this blog file, I’ve broken down the organization of digital tools from a technological point of view. Unlike a previous dossier on nitrogen fertilization, the division into agronomic objectives was more delicate – with sometimes a little too much redundancy between digital tools. Here you’ll find the main functions of the digital tools:
- Observe and Measure: Data acquisition tools and instruments: these are mainly plant sensors, flow meters, soil probes and weather stations that measure and record the state of the system. These tools enable us to better understand the resource and/or agricultural practices (or, more broadly, human practices) and their impacts (withdrawals and discharges, water quality).
- Advice and support: Irrigation advisory and management tools and services. These are mainly decision-support services based on field data or on a wide variety of models (water balance) (multi-scale, complex system modeling). They also include optimization of plot irrigation systems and network management. In the long term, modeling tools are essential. They are both decisional modeling tools (i.e. current decision-making) and anticipatory modeling to cope with future climatic conditions (time projection and foresight).
- Manage and organize : Tools and portals for managing and making data available. This means not only providing access to weather data (farmers spend a lot of time checking the weather, after all) and water data, but also soil and agricultural parcel data. These tools enable knowledge to be disseminated and shared, and are useful for participating in water governance at different spatial scales (communication, collaboration and consultation).
- Act and apply : Digital tools for applying irrigation recommendations in the field. These tools are relatively little deployed compared with the other categories.
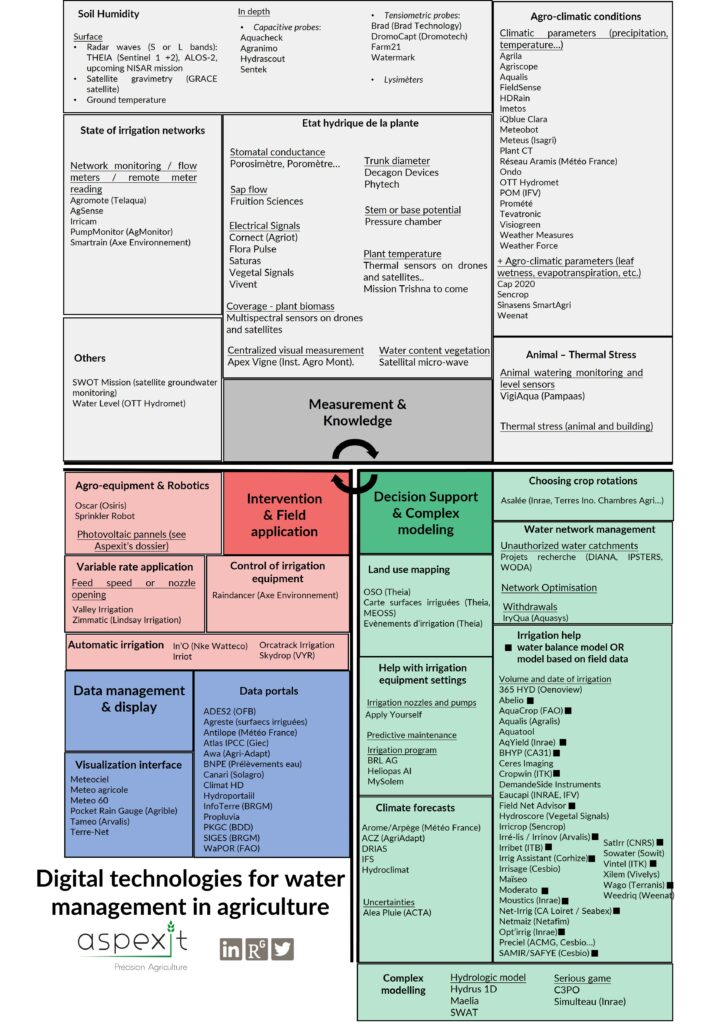
Figure 25. Digital technologies for agricultural water management (to be translated)
Observe and Measure
From an agronomic point of view, we can distinguish 3 main approaches to data collection using digital tools
- A “climate” approach: acquisition of meteorological data locally (connected weather stations) or on regular grids (often Météo France or European data reworked to generate agro-climatic data).
- A “soil” approach: acquisition of data on changes in the soil’s water status, in particular using soil probes (capacitive and tensiometric).
- A “plant” approach: acquisition of data on plant functioning throughout the season (evapotranspiration estimates, canopy temperature monitoring, dendrometry, stomatal conductance, sap flow, stem potential, thermal stress, etc.).
And let’s not forget flow meters and remote reading meters, which have a logistical and/or practical rather than an agronomic contribution to make.
Data can be continuous (acquired continuously) or discontinuous (acquired at given periods), local (stomatal conductance, for example, relies on the representativeness of a single leaf to represent the entire plant) or more global. As discussed above, the measurement of water in agriculture does not necessarily meet with consensus.
The CLIMATE approach
Access to large-scale climate data
Although many digital tools are proving their worth for water management in agriculture, the nagging problem will always be that of estimating the water that arrives from the sky – both in terms of quantity and spatial distribution. In agro-meteorology, the question is how to take into account variability at all spatial scales, and how to combine the different means available to obtain it (real and virtual data). A wide range of technologies are available for obtaining data on relatively large spatial scales
- Rain radar data (traditional and bipolarization radars)
- Satellite data for ground-based rainfall forecasts. These data are not always very accurate, but at least they exist when there are no other infrastructures.)
- Measuring the attenuation of electronic signals from geostationary satellites that are weakened by raindrops.
Geostationary satellites are preferred to circulating satellites for rainfall measurement because we can’t afford to have different chronicles of data.
In France, the best-known rainfall data are certainly those provided by météo France. Antilope data, obtained from a combination of radar data and rain gauges in the field (for spatial recalibration), provide climatic information at a scale of 1 square kilometer, thanks to atmospheric simulation models. Bear in mind that these data are dematerialized and virtual. While they are adjusted by field measurement points (rain gauges), they remain calculated data. These data are generally purchased and then reprocessed by agro-meteorological service providers to derive parameters specific to agriculture (such as evapotranspiration).
Although climate forecasts are improving year on year, the spatial variability of precipitation remains a thorn in our side. A distinction is made between global numerical forecasting models (with relatively large grids of several kilometers resolution) and local numerical forecasting models (with grids of the order of a kilometer).
The European Centre for Medium-Range Weather Forecasts (ECMWF) and Météo France both use the IFS (Integrated Forecasting System) numerical weather prediction system to produce medium-range forecasts of major synoptic phenomena, ultimately positioning depressions and anticyclones. It is currently the world’s most accurate weather model for medium-term forecasts over the next 3 to 15 days. The IFS system generates forecasts on regular 9km grids across the globe. Météo France, using a different configuration of the IFS system, called Arpège, produces forecasts on a 5km scale in France. Arpège is a stretched-mesh model designed to optimize computing power, with finer meshes at the scale of France and more relaxed meshes around it (the resolution is 24km in New Zealand, for example, with the Arpège model).
Météo France’s Arome model is a local numerical weather prediction model, with data produced five times a day on 1.3 km grids and forecast timescales of up to 48 hours. Note that the Arpège and IFS models can also produce data at finer scales (around 2.5 km) by forcing them.
The most difficult forecast is the short- to very short-term forecast, of the order of 0 to 6 hours. These forecasts have been improved thanks to the computing power available and the assimilation of radar data. The Arome model, for example, can be adapted for immediate decisions (0 to 6 hours), with very narrow radar data assimilation time windows for fine-tuning short-term forecasts. Previously, short-term data were often generated by extrapolating radar information (by looking at how rain radar displacements evolved). Until now, these methods have been limited because they didn’t really look at the evolution of rainfall convection. These methods are constantly evolving.
The main models used are deterministic forecasting models, i.e. numerical weather prediction models that simulate atmospheric behavior. Other approaches have been proposed to deal with uncertainty in weather, using probabilistic approaches. The idea is to produce a set of forecasts from a distribution of possible initial states, i.e. models for which the physics has been slightly perturbed to take account of the uncertainty in the description of physical processes (e.g. sampling of initial errors with perturbations, sampling of modeling errors with perturbations in the physics, sampling of edge errors, etc.). The PE-IFS model generates forecasts at a resolution of 18 km (the resolution is coarser for computational and approximation reasons) up to 14 days ahead. Arpège forecasts, when produced within a probabilistic framework, are closer to 5-7 km spatial resolution.
Météo France data is still subject to payment and is not Open Data. Even though we are beginning to have fairly detailed data on French climate change thanks to DRIAS data (on an 8km scale), we could ask ourselves whether, in view of the climate emergency, Météo France data – which remains a public body – could not fall into the common domain so that all players working with meteorological data can refine their models. The quality of the data will necessarily depend on the scale at which you wish to work. On a regional scale, we are generally interested in assessing the current state and adaptive capacities of territories in relation to water management. At this rather large scale, virtual data are well suited. At the topo-climate scale (0-10 km resolution), i.e. a scale consistent with that of a farm, virtual data remain interesting, especially if the farm’s plots are fairly dispersed in space. At the finer scale of a plot, rainfall is not too variable, but virtual data are not really suitable (weather station data are preferable – see next section).
Access to local climate data
This section contains a few summaries from a previous blog entry
Weather stations connected locally on the farm can be used to characterize climatic demand and understand the memory effect of the site (a sort of climatic profile of the area over time). These stations provide contextual information and are of interest for a whole host of applications involving radiation balance (the gold standard remains eddy covariance – a radiation balance by measuring eddy covariance in several directions), saturation vapour pressure or wind speed (see the following sections on the Plant approach).
Weather stations can include several different sensors:
- Thermometer: for air and/or ground temperature
- Rain gauge: for cumulative precipitation. This is undoubtedly the factor you can control most easily (unlike relative humidity).
- Barometer: for atmospheric pressure
- Hygrometer: for relative humidity
- Anemometer: for wind speed and direction (gusts, etc.)
- Pyranometer: for solar radiation
- Dew point sensor or wetting sensor: for foliage wetting
- Capacitive probes and tensiometers: for soil moisture measurement
Not all weather station suppliers are manufacturers, but many are assemblers. In other words, they don’t build their own sensors, but rather buy them from sensor manufacturers and assemble them into a weather station. This is not a problem in itself, quite the contrary, but it is important to bear in mind that some suppliers may have the same sensors as their competitors. These assemblers will then be able to differentiate themselves by the type of specific sensors they can install on their station or sell alongside (leaf wetness sensor, dendrometer, probes and tensiometers…), the number of sensors they can install on their station or the ability of their acquisition boxes to integrate all types of sensors (including sensors you might have bought elsewhere). It may also be interesting to know whether the sensors sold are dissociable (with their own antenna and transmitter), so that you don’t have to place all the sensors on a station in the same place.
Should I install my own weather station or use data from a reference station? Should I install my own station using reference standards (e.g. Météo France standards) or not? This will depend on a large number of factors, including whether or not the reference station is close to the farm, whether or not soil and climate conditions are very heterogeneous on the farm, and also on the climatic parameters the farmer wants to monitor (not all stations are equipped with the same sensors).
Virtual weather stations also refer to extrapolations of forecast model data (see previous section) to fine spatial scales. So, is it better to choose between a real or virtual station? One might imagine that, if radar and satellite data were to improve as much as possible, we would no longer need ground-based infrastructures. However, given the current resolution of forecasting data and the variability of climatic phenomena, even at very fine spatial scales, we’re not there yet. In any case, local data will always be needed to calibrate large-scale forecasting models.
A real weather station actually measures what’s happening in the field, unlike data from a climate model (which needs to be properly calibrated against what’s happening in the field). We therefore need to look at the level of uncertainty that may exist between a weather station in the field and what is proposed by suppliers of spatialized climate data. If the calibration is good, the virtual weather station means you don’t have to worry about maintaining the station, since it doesn’t exist in reality. However, there will be a subscription or fee to pay for data retrieval.
In the case of a real weather station, you will of course have to pay for the station and potentially a subscription to use a mobile application or associated website. Bear in mind, too, that a virtual weather station won’t give you access to all the climatic parameters potentially accessible from a real weather station (leaf wetness sensor, etc.). The choice of a real or virtual station may also depend on the size of the farm. Larger farms may find it useful to use virtual data, perhaps combined with one or two field stations, to properly calibrate the data.
Whether you’ve set up your own station at home or not, the next question is whether there’s any point in joining an existing station network or setting one up. Is there any point in sharing climate data with your neighbors? Yes, certainly, if the exchange is on both sides, i.e. if joining a network gives you access to the climatic data of the people in that network. In particular, this reduces data acquisition costs and enables data to be shared within a community. An additional benefit could be to benefit from subscriptions to warning network services, based on climate data from station networks.
One of the complexities behind a network of weather stations is the harmonization of the climatic data passing through the network. If these climate data do not come from stations of the same brand and/or if the sensors are not properly calibrated, then how can you trust the climate data around you? It also depends on the accuracy of the data expected in the field. This harmonization also has an effect on what will be done with the data, particularly if it is integrated into a decision-support tool (DST), for example. Some weather station network owners go even further, separating the stations they own (i.e. those they manage) from those owned by farmers, potentially precisely because the data from certain stations has not been validated. This avoids the need for these station network managers to deal with the maintenance and upkeep of farmers’ stations.
Correctly positioning your weather station(s) on your farm is essential to ensure that the data collected is both representative and exhaustive. You need to be aware that the representativeness of the measurements will not necessarily be the same at the top or bottom of a plot, near or far from a hedge, or near the edge of a plot. Some experts also advise taking a keen interest in soil profiles, so as to have a well-known and representative installation zone. These soil variabilities can be highlighted using digital tools (soil conductivity/resistivity, satellite imagery), but also and above all on the basis of farmers’ knowledge of the terrain (stony areas, frost spots, etc.).
Station positioning can also be governed by standards, and these are not necessarily the same for everyone. Some may, for example, choose to install stations within the framework of météo France standards (with sensors at a certain height, in an open area…), but it’s important to be clear that the data is good where it’s measured (not all data is measured at the same height above ground level, for example). Between a station positioned in an open area and one set up in the heart of an orchard, conditions will not be the same. In the heart of an orchard, we’ll be much closer to the climate of the crop in place, and the climatic data will better reflect the situation. The major drawback, however, is that most agro-climatic models have been calibrated to French weather standards. Are the differences significant? Do we need to revise the OADs if the stations installed don’t meet French weather standards? Not necessarily all the time, but it’s important to keep these ideas in mind (so be careful when you start making more detailed analyses, for example with dendrometers and the like).
And these stations will need to be maintained throughout the season (by the farmer himself or by a dedicated department of the station supplier or operator) in order to take preventive action and avoid, or at least limit, corrective action. Logistical problems around the stations can take many different forms: battery problems, breakdowns and breakages of all kinds, sensor drift, wear and tear of parts, clogging of the rain gauge, storage of the weather station…
The PLANT or ANIMAL approach
Understanding water flow in plants
One way to study the impact of climate change on water use is to start at the leaf level. The effects of a change in CO2, water and temperature regimes will be most obvious at the leaf level, as there are none of the confounding effects of canopy or soil architecture on plant energy use. Changing scales, i.e. being able to move from the scale of the leaf to that of the entire plant canopy, is far more complex – and we can’t simply extrapolate results from one scale to another because one leaf isn’t necessarily representative of the entire canopy (the position and architecture of the leaf in the canopy plays a role, for example, in the photosynthetically active radiation (PAR) regime and the leaf’s water demand). Mutual shading between leaves and interferences between leaves become pre-dominant factors on photosynthesis and transpiration capacities. Bear in mind that radiative transfer is difficult to achieve on multi-stratum and multi-species (when the canopy is complex, of course).
Foliar energy utilization shows a distinct profile depending on the carboxylation pathway (Figure 26), i.e. C3 photosynthesis, C4 photosynthesis and crassulacean acid metabolism (CAM). Corn photosynthesis, for example, is C4, meaning that the first molecule formed has 4 carbon atoms. This C4 process is characterized by a higher photosynthetic yield and better water use than C3 plants. Maize, for example, produces an average of 40 kg of dry matter per hectare per millimeter of rainfall, while wheat produces 25 kg.
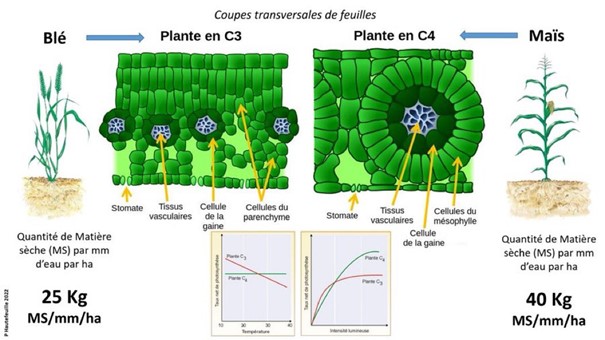
Figure 26. C3 and C4 plants
Stomata are the natural openings in plants that enable them to exchange gases (carbon, water…) for respiration and photosynthesis. When temperatures rise, plants tend to close their stomata to limit water loss. Closed stomata are not a sign of a stressed plant. Reduced exchanges will certainly mean lower yields at the end of the season, but stomatal closure is not necessarily water stress from a physiological point of view – rather, it should be seen as an adaptation or regulation of the plant to its environment. In any case, the relationship between lack of water and yield loss is not linear.
Plants have many ways of regulating their water. Stomata are certainly one of them, but plants can also change the orientation of their leaves (in relation to the sun, to create shade…) or allocate their resources differently within their organs. Genetic selection has helped to reduce plants’ drought resistance traits (in order to maximize production traits instead). Some selected plants have thus seen stomata appear both on the underside (as usual) and on the top to maximize gas exchange, but at the risk of increasing transpiration and making it harder to regulate. It should also be noted that, in addition to temperature changes, changes in CO2 levels in the plant’s environment lead some plants to change their stomata density too.
When the flow of water in the plant stops, so does the phloem pump. Elaborated sap becomes too viscous, and the plant’s ability to transport sap to the roots and reproductive organs becomes limited. These stoppages in water flow also lead to cavitation (air bubbles) in the plant’s canals. With these cavitations in place, if the plant is watered again, death is potentially assured, as the air bubbles remain in the canals.
There is a trade-off between water and nitrogen use efficiency in plants, making it impossible to maximize both simultaneously. And these trade-offs can clearly vary from one year to the next, depending on the conditions observed. This physiological dilemma at leaf level could be expressed as follows: given that the intercellular CO2 concentration in leaves is generally not saturating for carbon assimilation, any increase in this CO2 concentration would increase photosynthetic nitrogen utilization, but would also increase transpiration (Gong et al., 2010). The point here is that for photosynthesis to increase (and for the plant to convert light energy into chemical energy for itself), stomata must be open to transport atmospheric CO2 from the plant, but this is at the expense of plant transpiration.
Plants have an impressive ability to adapt to these water- or nitrogen-limiting conditions, by evolving their morphology and functional traits (leaf surface) and the nitrogen investment of the leaves (nitrogen concentration in the leaves, and leaf N content per surface).
High water productivity therefore requires an adequate supply of nitrogen. It’s important to understand that it’s a bit like using water and nitrogen as substitutable resources. When there’s a lot of nitrogen, the stomata don’t need to be wide open, because Rubisco – the key enzyme in photosynthesis – is working at full capacity and can photosynthesize with the little carbon that comes in (because the stomata are not wide open). Similarly, if the water supply is very high, stomata can be wide open, so even with little nitrogen, the plant can still photosynthesize. Bear in mind, however, that this substitution does not take place in the same proportions.
However, the relationship between yield and nitrogen input conforms to the law of diminishing returns, and nitrogen use efficiency therefore decreases with increasing nitrogen input. The effect of individual inputs such as water and nitrogen on crop carbon, water and nitrogen balances therefore determines a trade-off between water productivity and nitrogen use efficiency. Optimizing water productivity in some farming systems may require nitrogen doses that are too costly, too risky from an economic point of view, or not environmentally friendly. This is particularly important where the ratio between the price of fertilizer and the price of grain is high, in environments conducive to nitrogen leaching, or where biophysical, social, economic or infrastructure factors limit fertilizer use.
Observing the interactions between CO2, temperature and water regime is important for understanding water use in a changing climate. While increased CO2 may help reduce water deficit stress, it cannot compensate for increased heat stress, and may even be negative. The overall result is that water use efficiency decreases with increasing temperatures, but increases with increasing CO2 at each temperature regime. Another complicating factor is the change in air saturation vapour pressure with temperature change and the feedback effect on leaf temperature. The change in air temperature around the leaf modifies the leaf temperature and directly affects the water vapour gradient between the leaf and the atmosphere. Air humidity in response to temperature changes will have a significant impact on plant energy efficiency.
Water and carbon are also linked in the sense that the amount of water a crop will need, and the amount of carbon it will be able to leave in the soil, are directly linked to the amount of biomass produced. The level of carbon capture will also increase the water-holding capacity of the soil (see “Understanding soil water flows”).
Generally speaking, it has to be said that we currently lack knowledge of how the water cycle works in the plant. And this complexity is all the greater given that the water cycle interacts with the nitrogen and carbon cycles.
Evapotranspiration estimates and energy balances
Imagery comes into play here to improve the measurement of vegetation cover (cover fraction, biomass level, etc.) and thus modulate potential evapotranspiration (ETP – and in particular Kcb) as a function of plot cover (Figure 27 – see section on “the water balance method”).
As actual evapotranspiration is difficult to estimate, potential evapotranspiration – linked to actual evapotranspiration by a crop coefficient – is generally used (although it could be measured using lysimeters installed in the field, but this is not really feasible in operational conditions). Potential evapotranspiration corresponds to the atmospheric demand for water. Potential evapotranspiration is calculated in a more or less empirical or mechanistic way, mainly on the basis of temperature, and possibly radiation, wind and stomatal resistance, depending on the method. This gives rise to a number of formulas that differ from one another from the outset. These formulas behave differently in response to climatic projections, mainly following an increase in temperature of course, but also depending on the concentration of CO2, which has a strong influence on the stomatal resistance of plants (they will need to evaporate less water to capture CO2 if it is in greater concentration in the atmosphere).
Potential evapotranspiration is generally calculated using the Penman Montheith equation. This equation is all the more interesting as it has the merit of operating at different scales (leaf, canopy, canopy, etc.).
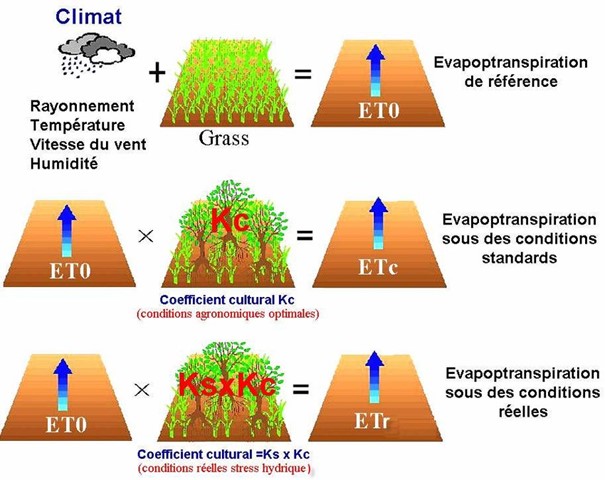
Figure 27. The FAO-56 method can be applied with a single crop coefficient (Kc) or a dual crop coefficient approach that separately represents soil evaporation (Ke) and plant transpiration and stress (Kcb and Ks).
The coupling of satellite data with water balance models (satellite alone is insufficient for field crops) provides another view of the plant’s water deficit, and is generally used subsequently to send irrigation recommendations (date or volume of irrigation). It is conceivable that the use of images could also be coupled with data on water quotas or restrictions in a given area. Integrated approaches involving models (hydrological and/or water balance) and remote sensing are an interesting prospect for further characterization of water in agriculture. This is the case, for example, of the SAMIR model, co-developed at CESBIO, which integrates the quantity of active vegetation (generally NDVI) into the FAO water balance model to improve the estimation of evapotranspiration.
The multi-wavelength synergy that we expect to see with future constellations will enable us to go ever further in the finesse of water balances and recommended water recommendations.
Canopy surface temperature monitoring and energy balances
By modeling the plant as a resistor, satellite or drone imagery data (particularly in the thermal wavelength range) can be used to calculate an energy balance. It is then possible to calculate indices of water stress or vegetation health, by calculating indices over various time periods (on a daily scale, or on longer time scales to detect drought). Approaches based on thermal satellites have been around for a long time. However, they have languished over time because the spatial resolution of thermal data was too coarse (Landsat resolution was not sufficient for agricultural areas, nor was temporal repeatability).
Thermal cameras mounted on drones, aircraft or satellites measure the temperature of vegetation cover, either directly or indirectly by measuring the temperature of the ground surface. These cameras use specific wavelengths of the electro-magnetic spectrum (i.e. thermal infrared). Since half the water a plant evaporates turns to cold, the assumption is that the plant’s temperature should be slightly lower than that of the air. If this is not the case, it is assumed that the plant does not have enough water.
It’s important to understand, however, that thermal imaging cameras do not provide a radiation balance at plant level. Wind conditions are often not measured. And wind often has a greater impact on plant water stress than temperature as such. Thermal stress is not necessarily a sign of water stress – the plant is just as capable of regulating the flow of water within itself. Canopy temperature reflects the plant’s ability to regulate its temperature and, ultimately, not much else; it doesn’t really tell us anything about the plant’s water requirements. And this capacity is linked to the plant, soil moisture and past practices. In addition, the water absorption peaks we observe in a plant’s spectral signature around 1400 and 1900 nanometers are not direct information about the plant’s water content, because water is considered to be saturated in the plant.
Generally speaking, approaches based on energy balances are rather fragile and the results are always very noisy. Models based on classic vegetation indices (NDVI type) do have their shortcomings, but they emerge with behaviours that can be fairly consistent with field data. With thermal approaches, the signal is noisy because the energy balance has to be made at the level of each individual image, with the associated assumptions and errors (depending on the types of radiative forcing used, for example). Over the past few years, satellite operators have taken it into their heads to extend the use of thermal imagery to agricultural monitoring. Examples include the future Franco-Indian Trichna mission and the LSTM satellite (thermal repeatability).
Water stress and water content in vegetation
The monopoly of measurement generally falls to Scholander’s pressure chamber method, which focuses on the study of leaf or stem water potentials. These measurements, which are destructive to the plant because leaves are detached and embedded in aluminum, can be taken just before sunrise (for leaf potential, which represents the balance between the plant’s water status and that of the soil) or in full sunlight (for stem potential, which represents the plant’s capacity to retain water when the foliage transpires). The pressure chamber evaluates the level of pressure required for a drop of sap to emerge from the petiole. The longer it takes for a drop of sap to emerge, the more water-stressed the plant is.
This measurement is rather time-consuming, since it has to be carried out leaf by leaf, and requires constant monitoring of the arrival of the first drop of sap. However, depending on the method used (stem or base potential), the data may not necessarily be representative of the entire plant.
Plant water deficits have two origins: a deficit of water vapor in the air and a deficit of water content in the soil. Saturation vapour pressure (SVP) is a way of quantifying the violence and frequency of heat waves. It’s a kind of suction pressure whose origin lies in a deficit of water vapour in the air. It can be defined as the difference between the quantity of water present in the atmosphere (or “actual vapour pressure”) and the quantity of water the atmosphere could contain at saturation (100% humidity, or “saturating vapour pressure”). And this VPD is one of the main forces governing plant transpiration. The warmer the air, the more water vapour it can store, so naturally, saturation vapour pressure increases.
The pressure chamber measurement represents the last link in the water chain from the soil-root interface to the point where the water is absorbed. It is therefore particularly sensitive to the pressure exerted by the atmosphere on the water.
Plant sensors, on the other hand, aim to measure water status as close as possible to the plant. If we don’t know how the plant works, we can reasonably assume that it will be difficult to do things properly. Water flow, for example, is important not only for photosynthesis, but also for regulating plant temperature. To convert plant sensor data into a physiological index, we need to know how the plant interprets the water deficit in the atmosphere and the water deficit in the soil. Note that plant sensors are generally coupled with atmospheric data (climate data).
These plant sensors can take several forms:
- Some plant sensors, for example, measure sap flow in a perennial section (of a vine) by measuring temperature differences on either side of the vine arm following local heating. This data needs to be interpreted at the start of the season and at peak transpiration times, in order to assess a percentage of hydric comfort.
- Other sensors are interested in the evolution of electrical signals in the phloem, the tissue that conducts the sap elaborated in the plant. These electrical signals are due to transient depolarizations of the cell membrane in the plant, caused by whatever is happening in the plant (pests, abiotic stress, etc.). Pairs of electrodes are used to measure various types of electrical potential differences (action potentials, variation potentials, system potentials) correlated with a particular water status.
- For perennial crops, particularly arboriculture, connected dendrometers are emerging, enabling real-time monitoring of the diameter of the stems and branches on which they are installed. This is an indirect measure of the plant’s water status which, depending on the day’s climatic amplitudes (humidity, temperature…), will fluctuate the flow of water passing through it.
Some crowdsourcing approaches have also been proposed using mobile applications. This is the case, for example, with Apex Vigne, which characterizes trends in vine water status on the scale of small regions and large Mediterranean areas, based on monitoring the cessation of vine growth (Apex method). Although growth stoppage does not necessarily indicate a lack of water, the authors of the application consider water to be the limiting factor for growth in Mediterranean conditions. The use of crowdsourced data nevertheless raises questions about the quantity of data likely to be retrieved, and its geographical and temporal distribution. These crowdsourced data will be used to invert water balance models.
We could also imagine vegetation water content being accessible with microwave imaging, because signal backscatter in different polarizations of cell membranes could be a proxy for plant water content. This would be similar to plant sensors. Some more pragmatic approaches attempt to link vegetation indices to field measurements (such as stem or base water potentials) and use satellite images to map stress states over wider spatial cover (and when we can’t instrument each plot individually). Imagery is particularly useful in areas where crops are not synchronized, and can be used to show the great heterogeneity of crop development.
Thermal stress in animals
Several digital tools can be used to monitor heat stress in animals. Even if we agree that heat stress is not water stress (we’ve talked about this for plants), monitoring heat stress is still, for animals, a way of taking an interest in their state of health. In particular, respiration rates, panting scores and micro-climatic conditions can be monitored at close quarters using climate sensors, various accelerometers (pedometers, collars, etc.) or vaginal probes.
In buildings, zones of comfort or thermal stress – which can be very heterogeneous within the building (due to fan settings, for example) – are potential stress amplifiers for animals. Thermal cameras can measure temperature or heat indices and map these indicators within buildings.
A number of connected drinking fountains will also be available to monitor water consumption by livestock.
The SOL approach
Understanding water flows in the soil
Soil is certainly the most misunderstood and neglected compartment in agricultural education, even though it plays a fundamental role in agricultural production. We could discuss soil from many different angles, but here we’ll concentrate on its role in the water cycle. When it comes to water, soil regulates the surface water regime and groundwater recharge. It determines the balance between runoff and infiltration of precipitation, and acts as a buffer against hydrological flows, helping, for example, to dampen flood phenomena or sustain river flows during periods of low water. On the agricultural side, the soil acts as a water reservoir, with fascinating capacities for infiltration and water retention, which we’ll discuss in greater detail later. These characteristics help to regulate the water cycle at plot level and throughout the watershed, cushioning rainfall surges and limiting erosion of the soil’s surface layer.
Plants are able to drink water from the soil if it is available. The usable reservoir (UR) is the amount of water plants need to grow. On closer examination, this reservoir can also be divided into two sub-reservoirs: the easy-to-use reservoir (RFU) – also known as the water comfort zone – and the difficult-to-use reservoir (RDU) – known as the water stress zone. When the easy-to-use reservoir is empty (because the plants have absorbed everything, or the water has evaporated or leaked into the environment), the plant begins to enter a state of water stress, in the sense that it can no longer easily draw water from the soil, and thus potentially no longer has enough water to cover its needs and develop properly. This first threshold is known as the temporary wilting point, because the plant can return to a state of hydric comfort if the useful soil reserve fills up again. If the hard-to-use reservoir also empties, the plant reaches its permanent wilting point, and then, clearly, it’s certain death. You can also see from the diagram that when the soil is saturated with water, plants also die of root asphyxia – the field capacity threshold is exceeded.
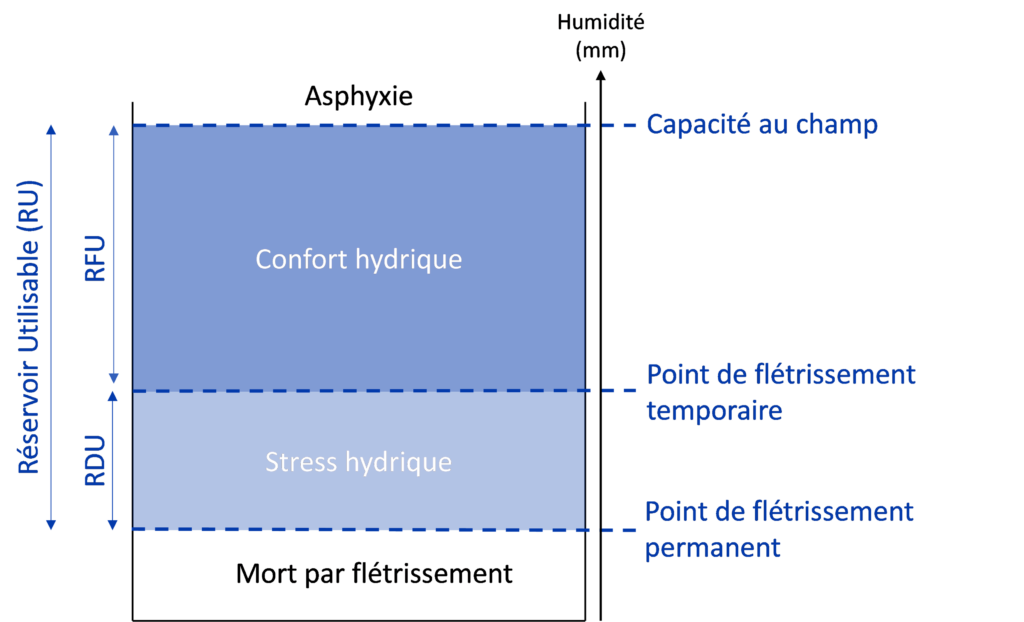
Figure 28. Soil water reservoir states for plants.
The size of the soil water reservoir usable by the plant depends on the plant and its ability to explore the soil with its roots. As plants grow, the accessible reservoir increases as the roots develop. So it’s important to bear in mind that this wilting point is not a constant – it can vary over time. Bear in mind that plants store very little water in their organs.
The size of the soil water reservoir usable by the plant is one of the main parameters used in crop models to simulate soil water status and its effect on crop yield. This fundamental parameter is often poorly known and difficult to estimate, as root conditions and plant rooting depths are not always well known. This useful reserve is generally set by default according to some general characteristics of the soil used (soil nomenclature, texture…). More than a reserve, it’s actually the plants’ capacity to absorb water from the soil that really interests us (we’ll come back to this in the section on digital tools for measuring soil water status).
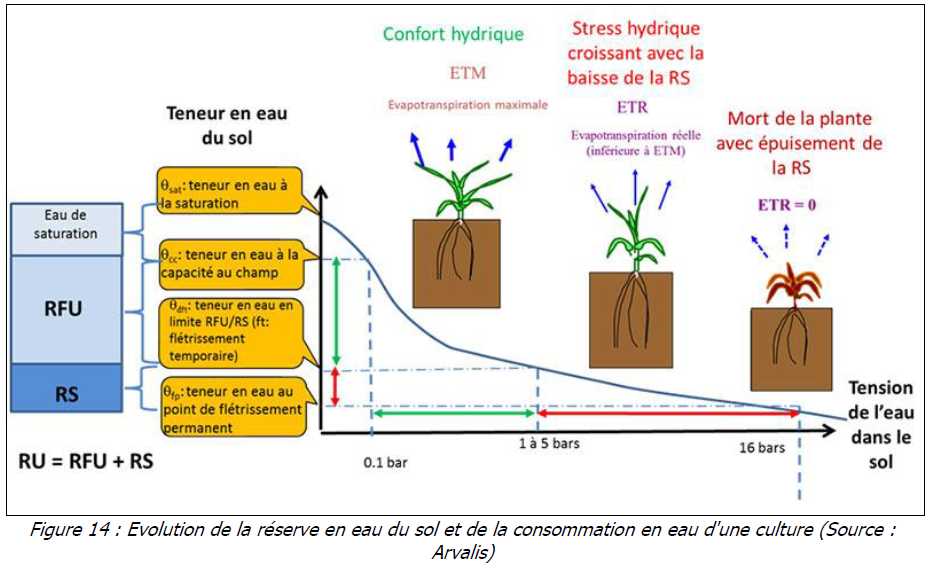
Figure 29. Evolution of soil water reserves and crop water consumption. Source : Arvalis.
Soil capacity to store water varies from one soil to another. This parcel-by-parcel and intra-parcel variability in the size of the water reservoir usable by plants depends on a number of parameters, including geology and relief, which influence soil depth, and soil texture, structure and fraction of coarse elements. Plant sensitivity to irrigation is also strongly linked to soil depth. In the case of deep to medium-deep soils, irrigation water is only used during the period of greatest sensitivity, provided the soil’s water reserves are full to begin with. For shallow soils, irrigation is efficient on yield throughout the sensitive period, although its impact is less marked at the beginning and end of the period.
Let’s continue exploring the subject of soil water reservoirs. We have seen that not all water in the soil can be used by plants, and this is particularly true of water of constitution and bound water (Figure 30). Water of constitution (or absorbed water) is that which enters into the chemical composition of soil grains and which is de facto not usable by the plant. Bound water (or adsorbed water) corresponds to the thin film of water surrounding soil particles. This water is bound to the soil by molecular attraction forces, making it unusable by the plant. The rest of the water in the soil is free water (or interstitial water) which, as its name suggests, is still more likely to be recovered or to move around in the soil. Water that can be used by the plant (the easily usable reserve) is that which remains fixed to the soil. This is capillary water, subject to the forces of tension developed by contact with water, air and the action of gravity. The rest of the free water is saturated water, which flows by gravity towards the water table when the soil is no longer able to retain more water than it already does. If water is no longer able to infiltrate the soil towards the water table, it’s because the soil structure doesn’t allow it (which is why we end up with root asphyxiation and/or heavy runoff).
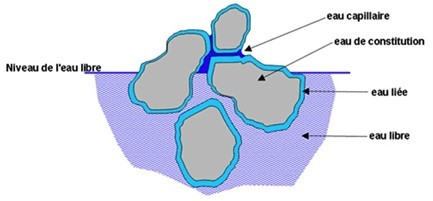
Figure 30. Different forms of water in the soil compartment.
How is water able to circulate in the soil? And how does the soil actually play its role as a water reservoir? Most soil water is stored in the smallest channels or pores in the soil – the mesopores and micropores. These tiny channels, which stick and store water against the pore walls in the form of films, are virtually only created by soil biology, namely bacteria, amoebae, mycorrhizae and the finest plant roots. Some soils are minerally capable of making holes (clays, for example, swell when it rains and deflate afterwards), but this is anecdotal. These holes in the soil, poorly joined, serve both to slow the flow of water and to retain it. Please understand that it’s the small pores that retain the water and give the soil its spongy properties. And this microporosity can’t be created mechanically with tillage tools… The larger holes created by the soil’s macrofauna (earthworms and other cousins) are obviously also important for soil aeration, but they are above all drainage channels through which water flows to prevent excess or hydromorphy.
To be functional over time, soil pores need to be stable. And this stability is provided by the soil’s organic matter. Soil architecture is maintained by organic glue, or in other words, by the carbonaceous materials released into the soil by plants. This organic matter is also hydrophilic, attracting water. Soil microorganisms thus maintain the soil’s architecture with carbonaceous materials that plants release into the soil. It is therefore by promoting photosynthesis, which ultimately feeds many microorganisms that in turn create space to store water, that the soil can play its fundamental role as a water-rich sponge.
Let’s not forget the fundamental role played by plant roots in soil microporosity. Most roots are made up of very fine, tiny roots, and it is these that dig into the soil. During their lifetime, roots release secretions into the soil – known as rhizodeposition – which, in addition to modifying the bacteria present in the soil, release organic matter into the soil and thus contribute to its architecture. At the end of their life, the roots die and are eaten by soil micro-organisms. The space initially taken up by these roots then becomes a new porous space in the soil. Generally speaking, roots are fascinating enough to explore the soil, sometimes to great depths. Trees, for example, can increase the volume of water available because the area of soil explored is larger. Deep rooting enables a water elevator mechanism to be put in place, i.e. a process that allows water to rise to levels of the root system that are in the presence of drier soil. In addition, some of this water feeds surface mycorrhizal fungi, which are potentially connected to other plants rooted superficially, thereby supplying water to neighboring plants.
When we plough the soil, we create holes. But these holes are actually of very limited interest. The holes are already large (so they’re not microporosity) and they’re not stable (since they’re not stabilized by organic matter), so they collapse. Ploughing also increases the respiration of soil micro-organisms, which in turn reduces the amount of organic matter in the soil, because the micro-organisms are put to work. As this organic matter serves both to bind the soil together (to solidify micro-porosity) and to attract water from the soil, we quickly come to understand the limits of ploughing on water storage in the soil. Let’s not forget that ploughing also destroys the soil’s mycorrhizal network, and we’ve seen just how important a role fungi play in watering neighbouring plants.
As you will have gathered, if there’s one player who must become the cornerstone of the strategy for adapting agriculture to climate change, it’s the soil. If you’d like to find out more, I recommend reading or listening to Marc André Sélosse’s popular work, it’s just fascinating
Soil water status
Soil water is measured in three different ways:
- via tensiometric probes that measure the force of water suction by the roots, i.e. the tensile force exerted by the root system to extract water from the soil
- Capacitive probes measure the amount of water present in the soil. These probes, with a single sensor or multi-sensors along a vertical soil profile, measure the moisture contained in a volume of soil and not on a surface in contact with a sensor. These probes can reveal soil structure, highlighting areas of compaction, for example, where water does not circulate. Capacitive probes can be fixed, for continuous measurement of water in the soil, or mobile – in which case the probes are moved between tubes already installed in the soil.
- Via the rarer lysimeters, which measure exactly what the plant uses
- We could also talk about probes that measure soil porosity to prevent root asphyxiation (in the case of excess water).
Soil probes are mainly used to check whether the soil has sufficient water content at the start of the season. Some consider that these probes cannot be used for irrigation, but only to assess the level of soil filling, because knowing that a wet front is present several tens of centimetres underground does not necessarily indicate what the plant needs.
The positioning of probes needs to be carefully considered, given the considerable variability of soil conditions. Placement must be considered in terms of spatial location within the plot and in relation to the irrigation system, proximity to crops (close to roots or not), and position in the water tower (rather at the beginning).
Sensors are limited by their installation depth. In the event of roots digging deeper than the position of the sensor, you may end up with slightly biased information. They may also be criticized for seeing less than the soil’s moisture bulb. Soil texture (presence of sand or stones) must also be carefully considered.
Water measurement in irrigation systems
Remote reading meters would provide access to detailed, real-time data on the volume of water withdrawn by farmers, which is still a major unknown at present. Without this measured data, estimates are still possible (for example, by working with irrigated areas and saying that such and such a crop requires so much water), but above all very uncertain. Some farmers also have their own boreholes, and it remains difficult to know what is actually drawn from them (see the section on “Irrigated and rain-fed farming in France”).
Manage and Organize
The data sources – which can be downloaded free of charge or viewed on platforms – are quite varied. You’ll find everything from :
- Climate data of varying degrees of aggregation (e.g. Météo France, Climat HD)
- Information systems providing water statistics on relatively large scales (FAOSTAT, Water STAT)
- Projections and adaptations to future climatic environments (e.g. Solagro’s Canari tool or Agri-Adapt’s Awa tool, FAO’s Wapor tool)
- Inventory and emission factor databases on the subject of water water (e.g. EcoInvent inventory)
- Data on water-related risks (e.g. World Resources Institute’s Aqueduct tool – Figure below):
- Data on drought conditions (European Drought Observatory, ProPluvia maps)
- Data on water withdrawals (Banque Nationale des Prélèvements en Eau, BRGM InfoTerre, departmental territorial directorate or chamber of agriculture databases, etc.).
- Data on the state of groundwater and rivers (groundwater monitoring via the ADES2 portal of the Office Français de la Biodiversité and the SIGES portal of BRGM, monitoring of river height and flow on the Hydroportail).
- Data on agricultural practices to assess the link between the state of water resources and what is happening around them (data from the agricultural census, the graphical parcel register, agricultural practices in field crops [PKGC], Terre-Lab data, etc.).
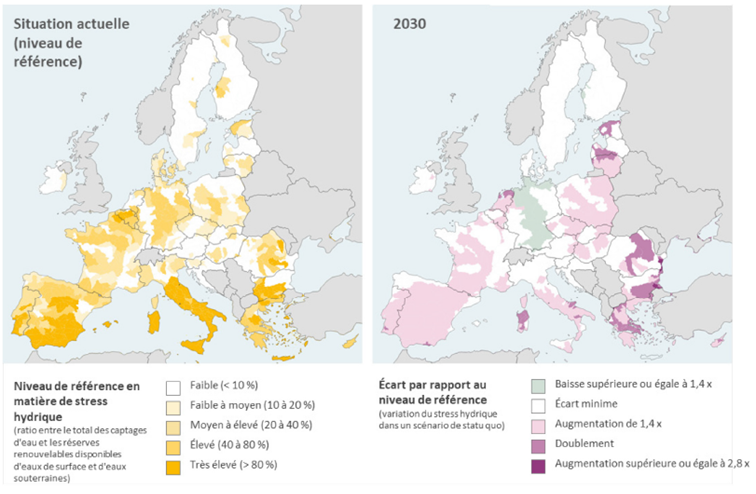
Figure. Projected current and water stress levels. Source: Aqueduct tool, World Resources Institute
However, not everyone has the same definition of open data. For some, for example, data can only be viewed and downloaded. Even if the reasons given may revolve around data anonymization (data can be aggregated at large spatial scales), the arguments are sometimes a little febrile. Data is sometimes made available several months or even years after it has been collected, which may limit its relevance for developing industrialized services (PKGC data, for example, is difficult to access, and the graphical parcel register is often available 1 or 2 years after the current year).
Météo France data is also subject to a charge, and we might ask whether, in view of the urgency of climate change, the data should not be considered to be of general interest and widely shared. This opening up of data could enable service providers to use these data in agricultural forecasting tools and/or complex models of agronomic situations.
More generally, although hydrological data are collected in almost all the world’s major river basins, their quality, distribution, availability and accessibility are highly variable, as are the variables monitored.
Setting up digital infrastructures around water knowledge (we can also talk about the digitization of water management) makes sense if stakeholders are to share their databases on common frames of reference, so as to be able to take a step back and make complex decisions by crossing and mixing multi-actor, spatial, temporal and multi-factorial databases. Each player still has its own data source and its own challenges. Modeling platforms are many and varied (hydraulic, hydrological, water-soil-bearing, groundwater) and are not yet interoperable.
In France, the dissemination, interoperability and standardization of water data are managed by organizations such as IOEau, with standards such as INSPIRE and SANDRE.
Consulting and Training
The water balance method
The water balance is established on the scale of the growing season, based on the principle that overall water inputs (rain, irrigation, initial soil stock) are divided between crop transpiration and the various losses: direct evaporation and spray drift, drainage, water remaining in the root zone after harvest and soil evaporation (Fig. 31).
The water balance equation allows us to check that the overall water balance established over the irrigation season is well balanced:
Rain + Irrigation + Initial soil stock = Transpiration + (evaporation/drift + drainage + water remaining in root zone after harvest + soil evaporation)
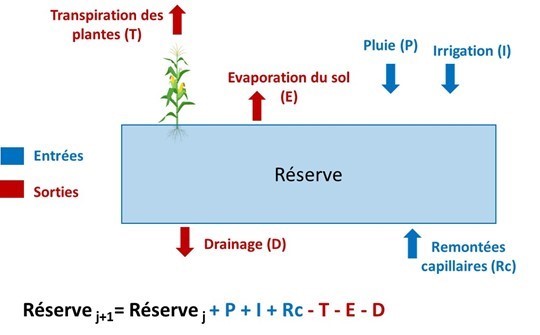
Figure 31. Inputs and outputs of a conventional plot-based water balance
While the balance method is now fairly well theorized and scientifically accepted, the difficulty in using the method lies in accurately estimating the terms of the equation. For irrigators, you may have guessed that the problem is to evaluate as accurately as possible the input (Irrigation) of the water balance that can be found once all the other parameters of the balance have been estimated. The decision to irrigate or not will thus be adjusted according to the initial parameters (soil type, size of useful reserve, crop, etc.), the real-time acquisition of meteorological data (rainfall, potential evapotranspiration, temperature) and the input of previous irrigation water.
The water balance method is one of two major irrigation control methods. Unlike methods based on measuring soil parameters (see “Soil water status” section), the water balance method is a form of soil-plant-atmosphere system modeling.
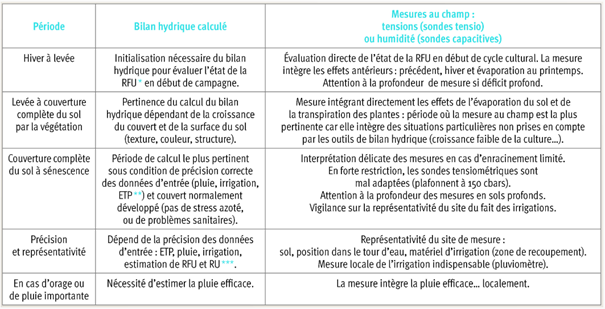
Figure 32. Advantages and disadvantages of the two main families of decision-support tools for irrigation management. Source: Gendre, S. (2020). Irré-LIS®, an example of an irrigation decision-support tool. Cairn.Info
The classic water balance models are shown in figure 25.. The FAO has also developed an open-access, royalty-free AquaCrop model, which is sometimes reused by suppliers of water management services. Other models (mostly used in research) incorporate water balance models and are used to estimate yield potentials and other water-limited yields (as we saw in the section on yield potentials): CERES, APSIM, or CropSyst
The water balance model is in fact a simulation of sap flow in the plant, but it has to be said that this flow is never actually measured. For annual crops, water status is rarely measured directly, because the digital tools for doing so are few and far between. Soil water content is often used as an indirect indicator of plant need. Instead of looking at demand, this approach focuses on supply. For perennial crops, measurement tools have already been proposed (sap flow sensors, dendrometers…). I invite you to reread the “Observe and Measure” section.)
The main limitations of these estimates of balance parameters concern useful reserves and plant evapotranspiration, notably because there is no simple linear relationship between soil water content and plant water requirements.
Depending on the situation, evapotranspiration can be the main component of the water balance, and therefore has a major impact on modelling (see section “Estimating evapotranspiration”). It is also the component most directly and strongly affected by climate change, on the one hand, and anthropogenic activities on the other (land use, cropping systems, irrigation…). A distinction is made between actual evapotranspiration (ETR) and maximum evapotranspiration (ETM). ETM is the maximum quantity evaporated by the entire crop, corresponding to the crop’s needs. ETM corresponds to the quantity of water (in mm) evaporated by the soil and transpired by the plant per day under normal growing conditions. I remind you that, as we saw in the section “Estimating evapotranspiration”, actual evapotranspiration is difficult to estimate, and it is generally potential evapotranspiration – linked to actual evapotranspiration by a crop coefficient – that is used.
One way of assessing the plant’s water comfort is to estimate the ETR/ETM ratio during the production period (particularly the flowering-maturing period for annual crops). The water deficit, or potential irrigation requirement, can be estimated from the difference ETM – ETR, calculated over the same period. These two values, water comfort and water deficit, can then be compared with supply (rain and/or irrigation) and climatic demand (potential evapotranspiration) on an annual scale.
On an annual scale, the state of development of the crop – its phenology – plays an important role in the plant’s demand for water. Global warming will therefore also have an impact on ETM via the shifting and shortening of phenological cycles. A number of factors will influence the evolution of ETM during the flowering-maturation period (the period when water comfort is calculated for most species):
- ET0 – reference evapotranspiration
- the cultural coefficient (kc), which represents the influence of foliage,
- the length of the flowering-maturing period and
- calendar positioning in relation to climatic demand.
The ET0 value at a given calendar date tends to increase with rising temperatures and radiation. However, this increase is held back by the antagonistic effect of CO2, particularly in C3 plants (wheat, sunflower, fescue, grapevine, conifers and broadleaves), due to their ability to reduce transpiration in response to rising CO2 levels. In C4 plants (maize, sorghum), this brake is not very active. The value of the crop coefficient can decrease under conditions of extreme drought during the vegetative phase, affecting the leaf area index, as can happen with rain-fed spring crops grown on superficial soils.
The crop coefficients (Kc) conventionally used are fairly generic. They are derived from aggregated literature, not always spatially localized, which can lead to errors in estimating actual evapotranspiration. According to some interviewees, crop coefficients are often overestimated in the literature, leading to irrigation demands that are too high in relation to reality. Estimating a crop coefficient is complicated because there are important relationships between plant architecture (width, height, porosity, orientation…) and its water balance. Each plant also has a different capacity to extract water from the soil through its root system (different rootstocks also have different levels of water extraction). These crop coefficients need to represent plant conditions as accurately as possible, and must be estimated at the time the water balance is taken (the crop coefficient varies over the season). For this reason, some studies emphasize the use of imagery (often satellite imagery) to refine the determination of crop coefficients.
Crop coefficients are currently defined within specific validation ranges. We are already wondering how they will evolve with global warming and rising atmospheric CO2 levels (we can expect, for example, accelerated crop scald). Crop coefficients are valid for relatively simple cropping systems (mono-specific stands). What happens when we start adding mixed systems or systems with different row spacings? Would these systems really reduce water consumption?
These balances can be established on larger spatial scales. In this case, we speak of hydrological balances – which tend to be drawn up at catchment scale. At larger water allocation scales (e.g. watershed level), there may be considerable uncertainty about the amount of irrigated land, the type of crop and, above all, the type of soil. Models such as Simult’eau (Inrae), Maelia, or Wasabi (from the ELSA PACT consortium) are used here. These are generally integrated watershed models that model water flows within a catchment area, with the aim of helping decision-makers, testing hydraulic development scenarios or agricultural options, or testing climate change scenarios. At these scales, the uncertainty linked to the crop coefficient remains fairly minimal – the bulk of the uncertainty resides in climate modelling (passage from a global model to a regional then local model) and in soil typology (shallow, deep soil…). The figures needed to construct large-scale water balances exist for each catchment area (evaporation rate, consumption by use, etc.), but there are still major approximations, notably because we only consider volumes withdrawn and known, whereas a great deal of water is withdrawn and returned to the environment.
Most irrigation tools work by asking for maximum evapotranspiration (ETM), so as to limit the risk of not meeting plant water requirements. It is therefore also implicitly assumed that the plant should reach its final yield without water stress. A first limitation of this way of thinking is that we’re going to have to review our use of water considerably anyway, and in all likelihood reduce it.
The question of irrigation needs can be examined in different ways: we can favor an analysis “at potential” which avoids any limitation of water supply to plants (ETR= ETM), or we can integrate a hydrological and agricultural reality which is characterized by a more or less marked unavailability of water resources (ETR < ETM). We can also distinguish the case of crops for which irrigation is central, typically maize, from crops for which irrigation only comes into play at the end of the cycle, typically wheat, or crops for which irrigation is supplementary, such as sorghum or sunflower. What we’re proposing here is a more refined agronomic approach and a rethinking of the notion of water efficiency. We might also ask whether yield is really the most appropriate parameter for thinking about water productivity. Perhaps yield should be replaced by a technical-economic indicator, or not an environmental indicator. We may even have to juggle several criteria at once – and weigh them up – before making an irrigation decision.
Calibrating water balance models remains relatively complicated. And from a physiological point of view, it’s not easy to know whether irrigation relieves plant stress or not.
A limited resource is not necessarily limiting every year. When equipment flow rates are drawn up, we finally know that we won’t be equipped to manage every climatic year, and that some years will be more complicated than others. Some tools are therefore beginning to develop the concept of limited-volume irrigation, in the sense that the volume available will not cover the plant’s water requirements every year (e.g. Irré-LIS from Arvalis). The Arvalis water balance model is first used on a plot with a long history and no quota constraints, to estimate the volume needed to be in ETM on the plot. Water quotas are then requested to switch to limited volume, and the tool recreates a curve of water destocking in limited volume. An available volume curve is constructed at the start of the season, and we then look at the dynamics this curve should follow to reach the end of the season, while distributing the volume as evenly as possible over the season.
Generally speaking, the tools can recommend an irrigation volume and/or an irrigation date. Some do not venture into estimating a volume, as they consider that the parameters underlying the water balance are not necessarily well known. Irrigation timing or scheduling is at the heart of irrigation strategy. Some service providers don’t actually propose a date on which to irrigate, but a date on which the crop will be under water stress, which then requires the farmer to recalculate the date on which to irrigate operationally. The irrigation date can also be found by testing water response curves on certain plots, using a sort of knowledge history to define the strategy that has proved most profitable in past years. The question of climatic frequencies being paramount – recommendations will become increasingly uncertain as climate deregulation progresses, especially as future climates will often be very different from those we have experienced in the past. These issues generally raise the question of the limits of current tools in the context of climate change. Current water balances are limited when it’s (too) hot, because they don’t yet take sufficient account of plants’ physiological limits.
Tools must be tailored to the farm’s irrigation strategy and equipment. A farmer with a gravimetric pump will not be in a position to control his irrigation in any great detail, and so may not need particularly sophisticated measuring or control tools.
Decision support for crop rotation in rain-fed and irrigated conditions
Water management can also be addressed through plot-based crop rotation. The Asalée tool, for example, compares rain-fed and irrigated crop rotation strategies, according to the volume of water available and climatic and economic variability.
Concertation support
We could also mention “serious games” in digital format – based on models and simulations – that can be used for consultation and discussion between local players in different spatial and temporal dimensions. These tools can be used, for example, to objectify water flows and discuss water sharing with various stakeholders. These digital approaches can also be used to study scenarios such as the impact of canal modernization, the evolution of agricultural practices if cropping and irrigation methods change, or the integrated management of surface water and groundwater in the context of climate change.
Support for the definition of agro-climatic zones
Climatic histories can be used to redefine production zones, as well as to more quickly identify years with climatic challenges for the purposes of exchanges with farmers (frequency of years with unfavorable climatic conditions, caused by these unfavorable climatic events, etc.).
Support for irrigation equipment adjustment
Digital tools also provide support for irrigation equipment settings. Nozzles, drippers, pumps and solenoid valves are all equipment which, when incorrectly adjusted, increase the differences between the recommended water and the water actually leaving the irrigation equipment. And the problems are numerous: poor pressure, sub-optimal application weather conditions, clogged nozzles…
These setting aids are already a way of avoiding unnecessary waste, but perhaps also and above all a way of ensuring that the recommendation matches the application.
Mapping humidity and irrigated areas
Satellite images – depending on their spatial, temporal and spectral resolution – provide a relatively broad and dynamic component for water management.
The first target is soil moisture mapping (volumetric soil moisture, especially at the surface). These maps tend to be generated by combining images from the active domain (radar imagery – particularly for soil information) and the passive domain (multispectral imagery – for vegetation information). And the algorithmic approaches used to build these maps are quite varied: physical models (e.g. Water Cloud Model), artificial intelligence through learning, coupled hydrological modeling and remote sensing approaches (with water balance-type equations to detect irrigation events, i.e. whether a farmer has irrigated or not).
Multispectral and radar information, which are not necessarily acquired simultaneously, are used to separate the influence of vegetation on the final signal reaching the ground (or put another way, the fact that vegetation attenuates the complete signal). Radar images can provide information on water content in the soil at depths of several tens of centimetres. C-band radar waves (6 cm wavelength) penetrate the soil by around 5-10 cm (this is the case with the Sentinel-1 constellation), while L-band waves (25 cm wavelength) penetrate slightly more (around 10-15 cm), but penetration remains low in agricultural environments with clay or loam soils. But it’s not as simple as all that. The water content itself already prevents the waves from penetrating too deeply. The same applies to soil depth and the type of crop being grown. In C-band, dense crops prevent proper detection of soil moisture. According to the interviewees, it would seem difficult to obtain information on soil moisture at depths greater than 15 cm with current satellite Earth observation sensors.
Radar imagery is quite complex to use, because radar data is impacted by both soil roughness and surface soil moisture. On a very rough plot of land after ploughing, there is often a tendency to over-estimate soil moisture.
Solving this equation with two unknowns (surface roughness and moisture) is complicated by the fact that today’s satellites only have a C-, X- or L-band radar sensor. This complex equation cannot be solved without making strong assumptions. We can hope that if the temporal repeatability of images improves, we’ll be able to couple images on the assumption that humidity or roughness hasn’t changed too much between the two data acquisition dates. However, this assumption is not always realistic. After ploughing, roughness will change and will not remain the same for too long, as the soil may have been reworked (resulting in smoother soil). Today’s semi-empirical models have been developed using large-scale databases to model the radar signal (as a function of instrumental and environmental parameters). Current physical models have been built with short-wavelength data (6 cm), but also quite a lot of L-band data. The smaller number of calibration points with long-wavelength data may raise questions about the transposition of the model with new data. Note that radar data will also depend on the angle of incidence and the wavelength used, but these instrumental data are known at the time of measurement.
The new NISAR satellite mission (3-10 m spatial resolution and 12 revisit days) promises to have both S and L radar bands, opening up the prospect of simultaneously addressing the problem of canopy penetration and correction of vegetation attenuation effects.
Current tools map surface moisture. And this surface humidity is different from the notion of useful reserve used in plant growth models. Useful reserve maps exist at scales of 90m resolution (but not at 90m precision). Going down to smaller spatial scales is far more complicated, not least because this useful reserve depends on soil texture, rooting depth and OM content – all soil hydrodynamic information that we generally don’t have at fine scales.
Nevertheless, there does seem to be a correlation between surface humidity and precipitation (but it’s not the same thing to go so far as to link it to climate change phenomena – work on larger spatial scales could make this possible). Moisture maps can be interesting for indirect information on rainfall. But it is perhaps irrigation mapping that is of greatest interest. Mapping irrigated areas is not easy, but it can be done. Detecting irrigated areas is a key issue, particularly in view of current events. It is mainly from the point of view of land use mapping, with or without irrigated zones, that the subject is dealt with. The work is going well for maize, where the hypothesis is that irrigated maize grows faster and stronger than non-irrigated maize (the work seems encouraging for wheat and sunflower, which are beginning to be irrigated, but the same mapping for perennial crops is more difficult.
What is far more complicated, however, is mapping irrigation episodes. Accuracy is quite good in semi-arid environments. On the other hand, in humid environments, especially when it rains, it remains relatively difficult to differentiate between rain and irrigation. In such cases, it is often necessary to take a step back and work on the scale of an entire basin to be able to separate these rainfall episodes. If radar signals increase on these large spatial scales, it may be possible to consider that rain has fallen. Irrigation system discrimination by radar doesn’t seem to be accessible either.
One way of mapping irrigation is to study the difference between radar signals from two different dates (forgetting about rainfall). At certain times of the year, there is virtually no error. But in summer, when the weather is very hot and plots are irrigated, soil moisture can change dramatically in just 2 days. In just a few days, the soil can lose its moisture and return to pre-irrigation levels. This means that the finer the temporal repetitiveness of the images, the better the chance of detecting irrigation episodes. Others will use multi-temporal images and map moisture anomalies over a year (without necessarily using model inversion).
Getting to the bottom of water quantities is another matter. Using visible imagery, we would ultimately have access to little information on irrigation water inputs. With thermal data (see below), we can begin to get a more precise idea of the plant’s actual evapotranspiration, and thus compare image results with water balance models to estimate whether water would have been applied. A certain amount of soil moisture could indicate whether or not water has been applied, but these methods are still rather laborious. So we’re still a long way from fine-tuned satellite irrigation control.
A number of projects are currently underway to produce land-use maps for early detection of summer crops. At national level, the OSO land use map exists, but does not always meet the expectations of managers who want to know the dynamics of crops at the time of the season and potentially in real time. The aim is to be able to estimate water consumption in a given area and its impact on flow rates. By coupling hydrological models with remote sensing images, the idea is to be able to monitor vegetation over the course of the year and refine low-water periods (by subsequently validating the models with remote sensing data in the field). Once again, satellite images are useful for spatializing phenomena that would be considered constant with hydrological models (for example, the state of corn growth over a large area).
Acting and intervening in the field
Some irrigation equipment is said to be slave-controlled in the sense that its operation is controlled by external phenomena. I’m not talking here about conventional remote control, which already exists as you might imagine for home automation control (remote activation of a pump, launching of a water tower at a programmed time) and which perhaps has more to do with electronics than with digital technology. I’m talking here about activating irrigation equipment in response to data from a nearby connected weather station, for example – a station that would trigger irrigation due to a lack of rain. Without going into too much detail, we could imagine insurance issues coming into play, because a farmer who loses his crop might want to complain about an automated system that failed to deploy irrigation in time.
It’s hard to find cultivation operations that can’t be modulated intra-parcel – irrigation being no exception. Intra-plot modulation originated in the United States, but is still used by very few farmers in France. Variable rate application can be achieved in two main ways: either by modulating forward speed (e.g. when there are several different crops – the system works for pivots and reels), or by modulating nozzle opening. Even if, technically, the infrastructures seem to work, we may well question the legitimacy of such installations, given that the variability of the parameters that should underpin modulated irrigation (useful reserves in particular) are generally not known at the spatial scale of modulation. Resistivity maps, coupled with auger sampling, could certainly be an option, but are not yet widely deployed.
The prospect of developing modulation is interesting for pivots, but much more complicated for the rest. On reels, with a motorized gun, intervention could be imagined on a half-hectare scale – far superior to that envisaged with a pivot. If we wanted to do the same thing with drip irrigation, we’d have to discretize the system and install solenoid valves everywhere to drive the device independently from one pixel to another on the irrigation modulation map. Solutions are being studied to propose dripper networks with lines controlled independently of each other. One interviewee preferred to use the term “precise irrigation” rather than “precision irrigation”, which he felt was less appropriate. Echoing a previous dossier on nitrogen fertilization , here again we find approaches based on the distribution of an average irrigation quantity over the plot and others based on the spatialization of an irrigation quantity (by which we mean the spatialization of a water balance over the plot obtained by modeling).
Generally speaking, the technical and economic benefits of irrigation modulation have yet to be proven. In any case, evaluation is complicated by the fact that, when looking at the intra-parcel scale, we can’t focus on a single factor. Even if irrigation equipment is already technically capable of opening and closing valves by irrigation zone (for modulation), the interoperability of irrigation data to integrate a recommendation map into irrigation equipment will still require a great deal of work…
Irrigation robots, still a relatively newcomer to the ecosystem, are beginning to make their presence felt. The French Oscar robot, which runs on hydro-electricity, is capable of providing modulated irrigation for crops less than 1m high.
Digital tools for “Action and Intervention” in the field also include modular solar panels, in particular those whose orientation is controlled by agronomic models, to limit crop evapotranspiration
Let’s take a step back
Do we really need digital technology to manage water?
Can we really measure and count everything? The example of remote meter reading
As early as 1992, the French Water Act made it compulsory to have appropriate means of measuring or assessing agricultural water abstraction. The meter (not connected at the time) provides data on the volumes withdrawn by farmers-irrigators over a season or a year. This data enables the user to position himself in relation to the use of his available quota and to draw up hydrological balances, as well as enabling the manager to better manage water releases and estimate what is in the reserves. Once connected, the meter provides access to daily consumption data rather than annual readings (often carried out manually), giving a more detailed picture of consumption in the field. For a manager, it’s also a way of better designing management and allocation strategies, and adjusting operating rules according to customer typologies.
Connected meters make it possible to compensate for conventional meters, which are not always accurate, and for declarative data from irrigators, which, in addition to not always being of high quality, can arrive with considerable delay. More generally, remote meter reading has several potential benefits:
- It is first and foremost a vector of knowledge, producing data to which certain promises of improvement will be attached. For example, the meter could be used for deferred water metering, to prevent irrigation quotas from being counted if the manager’s dam is not yet supporting the river flow.
- The connected meter can be used to detect leaks, particularly in water distribution networks or irrigation systems, and use this information for predictive maintenance. This is perhaps less of an issue in agriculture, since the price of water per m3 is lower than that of the conventional domestic network.
- Precise consumption estimates can be used to set up a kind of water exchange between irrigators – with the notion of water markets taking several forms (quota transfers between under-users and over-users). On the one hand, this proposal reflects the ambition to restore flexibility to the water-sharing system, in the sense that all available water volumes are allocated, but not necessarily used. On the other, the new measure enables managers to recognize water that has been appropriated and privatized by farmers in an informal way (outside of legal recognition), while securing a role in the organization of the water market by controlling sales (made possible by data).
- The knowledge provided by the meter could make it possible to reopen quota allocation by abandoning the reference to volume per hectare and surface areas subscribed in the past in favor of an overall volume defined at farm level (and taking into account the pedo-climatic contexts of each farm).
- Fine-grained data could also be used to adjust water pricing to the diversity of consumption, with the aim of reducing farmers’ overruns, especially in dry years.
- From a territorial point of view, the aggregation of personal water use data can be seen as a prerequisite for coordinated water management, with strong potential for anticipating and preparing for future crises (e.g. drought). In view of the growing stakes involved in water management, one could also imagine the strategic importance that such data could have, with certain organizations keen to sell this information at a premium.
- Some studies have highlighted the relevance of using this data to implement “nudges” or incentives to do better (one of the great advantages of these measures is that they cost almost nothing to implement). Messages are sent to irrigators, for example, informing them of their consumption and comparing it anonymously with that of their neighboring irrigators to encourage them, if their consumption is disproportionately high, to reduce it to the average level of their neighbors. These behavioral studies sometimes highlight unexpected rebound effects, in particular the fact that farmers judged to be exemplary raise their irrigation level to the average (instead of keeping their lower level). This rebound effect bears witness to the fact that water is the limiting factor on farms and that a farmer, when subject to a quota, will not simply refuse a portion of his volume if he uses less water than he is allowed. The tendency is rather the opposite, and the farmer will prefer to produce more with the same volume.
- However, network sizing is a fundamental issue for the manager (and network extension is very expensive and not necessarily accessible everywhere). This sizing is based on an estimated crop rotation in a given area, which in turn determines the size of the network and infrastructure pipes. With climate change underway and the need for farmers to adapt, crop rotation is likely to change rapidly, calling into question the size of the infrastructure. In this respect, digital metering may be a way of ensuring that networks are not oversized, precisely because it gives us a better understanding of farmers’ needs, and enables us to manage alert thresholds and network load shedding.
- Finally, the meter is also a means of preventing fraud, especially if it is connected and shares data in real time. It’s a way of ensuring, for example, that quantity restrictions at certain times of the year are respected (the rules can be different for each type of customer and for each peak period), that irrigation bans are followed, or to measure irrigation reductions of a few dozen percent.
A deeper question, however, is whether the meter is really envisaged as a management tool in the sense that digital technologies are not neutral (Collard et al., 2019). What data is actually collected? What water management paradigms are really underpinned by the use of this or that connected tool? What kind of data and indicators are put forward? How are these indicators constructed? Who really needs this information? Will detailed knowledge of the quantity of water used on a plot of land encourage water savings? Does the implementation of remote irrigation on a given perimeter necessarily lead to water savings?
Measuring and knowing how to count is not a trivial act; on the contrary, it is part of our relationship with the world. The act of measuring individual abstractions on a daily basis consolidates the desire of water engineers to have a say in the decisions with which they are involved, implying a reconfiguration of their relationship with farmers. In reality, we need to study and evaluate how the introduction of new techniques and/or technologies modifies water use and the more global landscape of water management. And we need to collectively break down this idea of digital objectivity, because tools are always built within a particular way of thinking. We need to produce an alternative discourse to technical subjects and paradigms, so as to resocialize and repoliticize water. Digital technology certainly provides information, but we need to ask ourselves what and who this digital data really serves.
The players pushing the use of connected sensors often have – sometimes hidden – reasons for putting them forward. If connected remote reading meters do indeed have the potential to facilitate the use and sharing of data, or to better manage scarcity issues, it is understandable, for example, that water distributors have a vested interest in pushing these sensors to acquire missing data to better calibrate their hydraulic models (and calibrate their water releases) and renegotiate flow or other thresholds with local players (to ensure better management of their water stock, avoid potential tensions, make sure they comply with the crisis flows they are obliged to manage).
The manager’s task is not an easy one either, since farmers can also exceed their quotas, which makes it difficult to comply with the low-water flow target, on pain of financial penalties for the manager. The use of digital readings inevitably casts doubt on the possibility of the readings being monitored by a kind of water police force. As far as water suppliers are concerned, it is indeed conceivable that institutional structures might require them to prove that their customers are not using their water indiscriminately, in the form of an obligation of means or results. Without going as far as copying, water distributors don’t really know all that much about how water is used after the supply point. They don’t know what crops are grown, or how much land is irrigated (or used in total), which in any case makes it impossible for distributors to assess the technical skills of their users (the farmers). And it’s all the more complicated when the hydrant is used by several farmers at the same time.
These issues show how the production and acquisition of water consumption data goes beyond a purely hydraulic ambition. This consolidation of hydrological expertise via the water meter is part of a reaffirmation of the manager’s legitimacy as a State-appointed water manager in this territory. The aim is to remain competitive in a field where the former concessions made by the State are no longer self-evident.
In this way, digital technology can bring with it the risk of a kind of delocalization or depoliticization of water. A bit like telecommuting, we might think we’re managing the problem from afar, and lose the desire to share and connect, and to understand what it means to have to use water collectively.
The meter transforms social relations, as its introduction is seen as an opportunity for water engineers to engage in dialogue with the relevant authorities to discuss the quantification of the river. The new measure and its intended use help to reconfigure relationships in which farmers-irrigators take on the role of users, or even customers, passive consumers of a resource because they are excluded from its management methods. The meter embodies the manager’s authority over the valley and his concept of good management. When the situation requires it, new arrangements are proposed to farmers-irrigators by the manager to give flexibility to the sharing system, with the acquisition of new data for the latter. To achieve this, the meter is staged and scripted, and becomes, under the action of the engineer, in turn a vector of knowledge (on daily abstractions), an object of consensus (to defer metering), of surveillance and partnership (to control possible sales), or even a conditionality (redefinition of the quota).
Digital technology certainly makes it possible to know water better, but the question we should be asking ourselves collectively is: who knows it better? If irrigation management, for example, is not based on daily monitoring of the quantities of water withdrawn, is the connected meter really that useful to the farmer? Is better quantitative knowledge more interesting than knowing the history of this water and its current management, where it comes from and how it evolves? Finally, automated meter reading can also be interpreted as a sign of the gradual disappearance of the technicians in charge of checking meters, considered to be the last link between farmers in the field and the water manager.
Data Reliability and Uncertainty
The quality of climatic data, and in particular forecasts, has improved considerably over time, especially data generated by Météo France’s Radar network, with significant work on improving calibration with ground-based rain gauges. Weather models use ever more input data to improve forecasts, and air traffic has played a significant role in improving models where there were no stations or radar (by taking sensors directly on board the aircraft).
As the number of digital tools for water-related issues increases, so do customer demands, which can sometimes give the impression that things aren’t moving fast enough. The biggest limitation is certainly the cost of improving accuracy (cost of calibration equipment in the field, equipment maintenance and data processing). The growing mass of processed data is helping to improve the quality of forecasts, but we are currently in a special context. Covid 19 has had a significant impact on the quality of weather forecasts, with the cessation of air traffic. Those involved in weather data had never anticipated that we would lose this source of information. In the space of a few months, the structures involved in weather forecasting regressed by several years in their ability to make predictions. We then continued to realize how fragile we were when it came to weather, with a poor grasp of many climatic phenomena (El Nino, La Nina).
Overall, scientific advances in climate and precipitation projections and their impact on hydrology are significant and ongoing. However, we still need to improve the accuracy of scenarios on a regional scale (downscaling), to enable those involved in water and agriculture to conduct forward-looking studies at local level.
The models are capable of several levels of forecasting.
- 3-day forecasts
- Beyond 3 days, we’d rather talk about trends than actual forecasts. We’re interested in trends when we’re dealing with instability (rain and water), and that’s not easy.
- Seasonal forecasts to assess temperature and precipitation trends for 3 months ahead.
Beyond a certain timeframe, predictability is no longer good enough, and probabilistic approaches are required – as deterministic methods are no longer reliable enough. Meteo France, for example, offers several possible meteorological models for this purpose: AromeEPS, Arpege EPS, IFS-EPS.
Uncertainties in climatic data can be presented in many different ways: quantile maps, gross probability maps (which provide information on whether a threshold has been exceeded and enable the spatial extent of a phenomenon to be targeted, but do not provide information on the dispersion, timing or maximum intensity of the phenomenon), probabilistic wind roses, normals and records maps. There is still a real gap in our understanding of the uncertainties and probabilities that lie at the heart of agro-climatic data. Even low probabilities are informative. Indirectly, we could say that farmers reason about uncertainties because they rarely look at just one meteorological source, even if the same subjacent models can in fact be found on the websites and mobile applications followed.
The digital tools used in agriculture (particularly decision-support tools) are sensitive to the quality of model input data (water balance on the subject of water and any other disease forecasting model, for example). There is sometimes as much uncertainty in the data as in the modeling (and it is not possible to instrument an entire watershed, for example). All estimates are valid for a certain type of calibration, and may or may not be valid for a given area – which means that data cannot always be extrapolated as is. The positioning of weather stations can therefore raise questions when the data from these sensors is used in climate models, often calibrated to French weather standards. For example, weather station data can be controlled at several levels: individual stations (verification of sensor operating rules, temporal gradients, simple meteorological assumptions and control of value ranges, interpolation of missing data), observation networks (control rules and study of consistency between several stations in the network, use of data external to the network using, for example, Météo France data and tools).
The use of fixed or mobile point sensors always raises the question of the representativeness and exhaustiveness of the data collected. In the case of plant or soil sensors, for example, the few measurement sites recommended are generally rather limited. An upstream sampling campaign will certainly optimize the location of these samples, but the coverage will be nothing like that of rain radar data.
And this uncertainty is necessarily propagated right down to the forecasting models used and the interpretation that must be made of the modelling results. The use of models (and therefore of AODs) must sometimes be reviewed in the light of calibrations, which can sometimes be significantly different. Real-time observation data assimilation systems can also be used to improve the local output of short-term forecasting models. Unlike disease models, water balance models are often run with rainfall totals, which makes them more resilient (in the case of a disease, the weather situation on any given day can totally influence the development of the disease). With rainfall totals, if we are potentially wrong on an estimate on a given day, the result may not be too affected if the data acquisition campaign is long enough.
The subject of sensor connectivity also needs to be looked at closely. In the case of connected meters, for example, it is problematic to have data that cannot be transmitted over the network. Some low-energy sensors, for example, fail to ensure that data has actually been sent, with the result that long columns of data can be lost – without being able to interpolate the missing data. As you can imagine, this is particularly annoying if these interruptions occur at times of high water abstraction. Rain can also cause signal attenuation, and curtains can clearly cut off communications (I refer you to a previous dossier on communication standards for connected objects).
Nor is digital technology the answer to all the ills of water management. It’s not because the technology is connected (in the case of a connected remote meter) that a collective network will be better managed, that irrigators’ decision-making will be good and that water use will be thought out intelligently on a watershed scale. Let’s not forget that water is heavy to transport, and that it entails major pressure constraints. The functioning of physical life in a water network is a real challenge. Digital technology and automation certainly have potential, but first and foremost, the system needs to open, close, detect leaks, etc. Automated or remote-controlled systems need to be capable of operating manually when required.
Studying water status is particularly complicated. The fact that many tools have a single-indicator approach should not be taken as a sign that the problem has been solved. Controlling perennial crops such as vines with tensiometric soil probes, for example, is somewhat questionable. Controlling vines during the veraison vegetation period using a probe is perhaps of little interest, whereas monitoring soil mineralization in winter using the same probes may be much more relevant. The advantage of digital technology is that you can choose from a wide range of sensors and data.
We still need to ask ourselves what level of precision is expected, before always wanting to dig deeper into the assumptions of the models used. In view of the propagation of uncertainty all along the chain – from data acquisition, to data cleaning, to integration in a model which itself is subject to uncertainties… – we can indeed ask ourselves to what extent the precision of the input data impacts the precision of the output data, and whether the decision taken at the end is likely to evolve? For example, what level of precision is needed to assess hydrological pressure, given the uncertainties of the hydrological modelling used?
Preparing for future water shortages
The cost of water in agriculture
The notion of sobriety is particularly complex to define and leads to debates that are difficult to settle: how far is it acceptable to put pressure on the resource? When is the alert level exceeded? This difficulty is all the greater given the shifting nature of the reference points, with climate change constantly shifting the cursor. What’s more, sobriety does not have the same value at all times or in all parts of the territory. When there’s an overabundance of water in winter, when the aquifers fill up or drain their overflow to natural outlets such as springs and rivers, what sense is there in talking about sobriety? Saving water is an imperative that is all the more consensual for its blurred contours.
The agricultural sector, a major consumer of water in the summer months, pays a water abstraction charge (excluding gravity-fed irrigation) set per cubic metre at a lower level than that imposed on drinking water utilities. Farmers pay less than 1 centime per m3 of water (slightly more for collective systems). The tariff as it stands is not yet a main driver for water savings. There is no real price signal for water. The only potential price signal is energy (because water management requires energy to pump water, for example).
Even so, the overall bill per farmer is not negligible, since irrigation can cost several hundred euros per hectare (including energy, labor and equipment), which is more than the average amount of aid per hectare under the first pillar of the CAP (Figure 35). Unsurprisingly, farmers consider water to be expensive. If the price of water were to rise, certain types of production could become unprofitable and be abandoned.
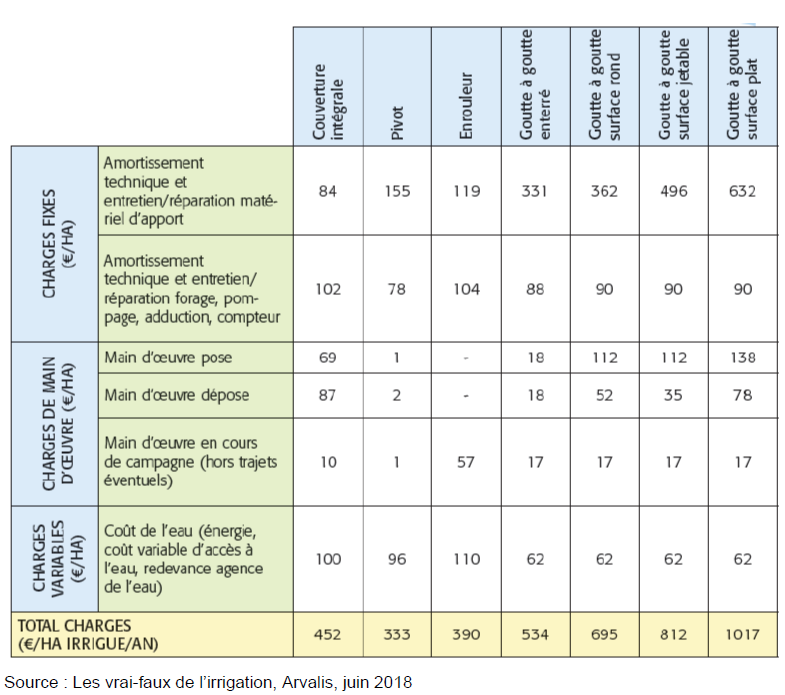
Figure 35. Irrigation’s true-faults. Source: Arvalis, 2018
In the end, it’s not so much the price as the scarcity or risk of running out that means water management can be transformed. A signal based on water allocation (for example, with an irrigation performance score to distinguish between good and less good irrigators, and give the former better access to resources) is relevant, but care must be taken to take account of the pedo-climatic contexts in place. Farmers with sandy or silty soils will have different water requirements. We could therefore imagine a stress test in the form of integrating water into the economic balance sheet of a farming business. The intention would be to objectively answer the following question: without water, what would be the farm’s overall turnover?
Without irrigation, certain markets and contracts would no longer exist. For example, in the case of beans, without irrigation, there is a risk of stringing and degrading product quality. In the case of tomatoes, we can expect that sizes will no longer be homogeneous without irrigation. Some crops are completely dependent on the volume of irrigation available in the summer. Water can also be a factor in increasing yield compared with the same crop grown under rain-fed conditions. Thus, the value of water to the irrigator can be approximated by the difference in value between an irrigated crop and a non-irrigated one, in relation to the volume of irrigation water required (possibly weighted by a drought risk factor). In France, there are no general, detailed studies on the value of water for different crops and production systems. However, such information could prove useful in defining arbitration and water allocation rules.
It appears that the water savings achieved are highly variable. For the same crop, this variability is observed between different trials, due to soil and climate characteristics (Serra Wittling and Molle, 2017). Within the same trial, results are not systematically reproducible from one year to the next. The greatest water savings are often observed in wet years, whereas they are less significant in dry years. Similarly, water savings tend to be greater in soils with low reserves.
The most widespread voluntary lever is the MAEC (Mesure agroenvironnementale et climatique), a CAP subsidy whose scope is limited, however, as it is based on an obligation of means rather than results. There is also the system of payments for environmental services (PES), with private or public partners remunerating farmers for actions to improve water quality.
Irrigators can claim European financial aid, from FEADER funds, for their agricultural hydraulic investments, provided that the new irrigation system is likely to enable water savings of 5 to 25%, with no reduction in crop yield. This aid is conditional on the irrigation technique used, which means that it is an aid for the acquisition of equipment (efficiency in terms of water saving).
Despite the quest for maximum value from irrigated crops, water resource enhancement systems often cannot simply be financed by irrigators alone. Costs can be quantified quite quickly (CGEDD, CGAAER; 2020):
- The investment cost of a replacement reservoir (in the form of a “basin”, with a geomembrane), with a filling and service network, is higher: around €6 to €8/m3 stored. Where water storage is envisaged (all types of reservoirs and dams), the cost of acquiring the land on which the water storage is to be built must be added. Despite the economies of scale observed with large-scale projects, we are increasingly seeing the construction of small-scale structures, which pose difficulties in terms of financial structuring, identification of motivating project owners and social acceptance (e.g. Saintes Solines), the environmental consequences of which are relatively poorly understood.
- Operating costs vary according to the type of reinforcement (e.g., higher energy costs for a basin transfer than for a reservoir) and the topographical context (pump head). They include both operating costs (energy, vegetation maintenance, management costs, etc.) and the cost of minor curative or preventive maintenance (pump replacements, minor repairs, etc.).
- Heavy maintenance costs: These costs also vary according to the type of resource reinforcement. They correspond to the renewal of structures at the end of their service life, and are therefore based on the different service lives of the various components of the structure (and also on the project owner).
And let’s not forget that some project developers are now insuring themselves against environmental protests.
Costs, both financial (operating and maintenance costs, cost of capital, administrative costs) and environmental (environmental damage caused by abstraction, storage and impoundment, opportunity costs related to other water uses as current and future users will suffer from a depletion of water resources), should be provided for and included in any economic analysis on the subject
Moreover, the Water Agencies cannot subsidize additional water storage projects that are not primarily aimed at saving water during the low-water period. Only the part of the structure corresponding to the substitution volume is eligible for support from the Water Agencies, up to 70% of the project cost (mega-basins, also known as substitution reserves, can be subsidized up to 70% by the Water Agencies). Maximum subsidy rates fluctuate between 40% and 80%, depending on the period and the source. This public investment subsidy, and in particular its rate, is important because :
- the overall cost to the project owner, and therefore to the irrigators, is made up of other items such as depreciation and operating costs, which do not benefit from any subsidies.
- the profitability of non-economic equipment is by nature negative.
- the inclusion of collective equipment costs in water pricing, and therefore the financing strategies chosen, have a very significant potential impact via the cost of water on the net margin of irrigated crops. Pricing can therefore be a very important factor in rebalancing to ensure equity between irrigators.
The long-term economic equilibrium of existing large-scale collective systems may be jeopardized by a reduction in irrigation in certain sectors. The development of resilience irrigation, for example (a form of water security as we saw above) for a proportion of crops, would require a specific program of public financial support. The economic equilibrium of this type of irrigation from a substitution reservoir would require that collective fixed costs be based on the mobilization of the entire stored volume (if resilience irrigation uses 4 times less water than production irrigation), pricing can only be accepted if there are 4 times as many irrigators), and that individual costs (amortization of the cost of the feeder pipe between the collective hydrant and the plot, and depreciation of sprinkler or drip irrigation equipment) be largely subsidized.
The economic study commissioned by the Union des Industries de l’Eau (UIE) puts the additional annual requirements for modernizing and decarbonizing water, wastewater and stormwater infrastructures at 4.6 billion euros. Funding levels, which have been falling steadily for the past 40 years, show a chronic investment deficit that makes it imperative to make water a top priority and ensure the resilience of our water services and infrastructures.
In general, the introduction of public aid or loans will be essential in order, on the one hand, to generalize technically controlled irrigation and the deployment of irrigation efficiency tools and, on the other hand, to cover the risks associated with the introduction of more water-efficient crops and practices over several years.
With regard to water used for irrigation, it is essential that pricing structures provide greater incentives for the rational use of resources and greater transparency with regard to competing uses, in order to avoid cross-subsidies from other sectors of society. The elimination of negative subsidies should also be a priority for future agricultural policy.
A necessary adaptation
We’re going to have to manage crisis situations. And the problem isn’t when things are going well, but when they’re not… At crisis level (the most critical), all uses are potentially subject to restrictions.
Understanding the notion of adaptation is fundamental, because we’re heading for disaster and we need to be prepared for it. The sensitivity of different regions to climate change is extremely varied in terms of the quantity and quality of water resources, and the risks associated with increasingly extreme events. Adaptation strategies must therefore be devised as close to the ground as possible, at the heart of this diversity of territories and sectors. The opposite of adaptation, maladaptation, hides in the details. If an adaptation action aggravates the problem it is supposed to solve, it is in fact a maladaptation action.
The notion of adaptation is at the heart of the debate here, and there are many forms of adaptation, from the simplest to the most advanced:
- Incremental” adaptation consists of adapting at the margin to a change of limited scope. The organization of the activity is not fundamentally altered. The use of irrigation is obviously one of the adaptation solutions, because in some cases irrigation will be an unavoidable solution, but it will remain a long-term minority in terms of surface area compared to the dominant rain-fed agriculture. Its evolution towards “resilient” irrigation, playing a securing role, is essential to optimize its use and enable better sharing of the available resource.
- Systemic” adaptation, when faced with a more profound change, acknowledges the impossibility of coping with it by adapting at the margins. The organization of the business is profoundly transformed.
- Transforming” adaptation involves changing the fundamental elements of the system in response to the climate and its effects, including new production and relocation. This includes far-reaching transformations of industries and social models.
Adaptation is a process characterized by uncertainty, as it is difficult to predict when adaptation is necessary and what level of adaptation will be required. Drought committees, for example, are exceptional in that they only interact when there is a crisis. However, it is necessary to adapt to situations that could arise. Beware, therefore, of short-term analysis of the benefits of implementing adaptation practices. Adaptation remains an iterative process that needs to be regularly questioned over time. We are engaging farmers in a long-term approach that will accompany the economic life of farms.
The water plan unveiled by the government aims to reduce water abstraction by 10% by 2030. However, it is important to understand that adapting to an average 10% reduction in water abstraction does not guarantee that, within this average, we will be able to adapt to a 25% reduction in water abstraction in a critical year.
The IPCC has proposed numerous adaptation options related to water and food security risks (of which water is a crucial input). Beware, however, that the effectiveness of adaptation options is mainly mentioned qualitatively, but not assessed, and the effectiveness of combinations of measures is rarely evaluated.
Here are a few examples:
- Varietal precocity adaptations reduce yield losses by shifting the cycle to a cooler time of year, and also limit the increase in irrigation water requirements, but reduce the period of photosynthesis and grain filling. Species selection and calendar shifts alone will in any case be insufficient to fully mitigate the losses expected from a warming of more than 3 degrees compared to pre-industrial times.
- Digital technologies are also a possible route to adaptation, as long as belief in techno-solutionism does not reduce the space for solutions and the timetable for adaptation options. It will be necessary to ask how digital tools can help us get through crisis situations, and to assess their potential in terms of adaptation.
- Assessment of irrigation requirements and the impact of CO2 and O3 (ozone) tends to focus on mono-specific species, making it difficult to extrapolate certain results to multiple stress factors and mixed and diversified production.
- On the livestock side, we could imagine a type of transhumant or service livestock farming, characterized by a revisited organization of work where the farmer would have a reduced land base thanks to the forage resources of neighboring farms.
- The transition, for a greater or lesser proportion of the farm – depending on the sector or region – to security irrigation profoundly alters the use and therefore the profitability of the corresponding investments. In order to be as flexible as possible, farm equipment will have to be totally or partially mobile, or at least more agile, to adapt to irrigation configurations that vary from plot to plot and from year to year, ranging from technically controlled irrigation to supplemental irrigation. This implies an improvement in mobile irrigation equipment, which today is generally less efficient than fixed equipment, and the acquisition of new mobile equipment for irrigators who currently only have fixed infrastructures. For collective irrigation distribution networks, the development of resilient irrigation means re-examining their economic profitability in the light of the lower quantities of water supplied per hectare, and not systematically every year.
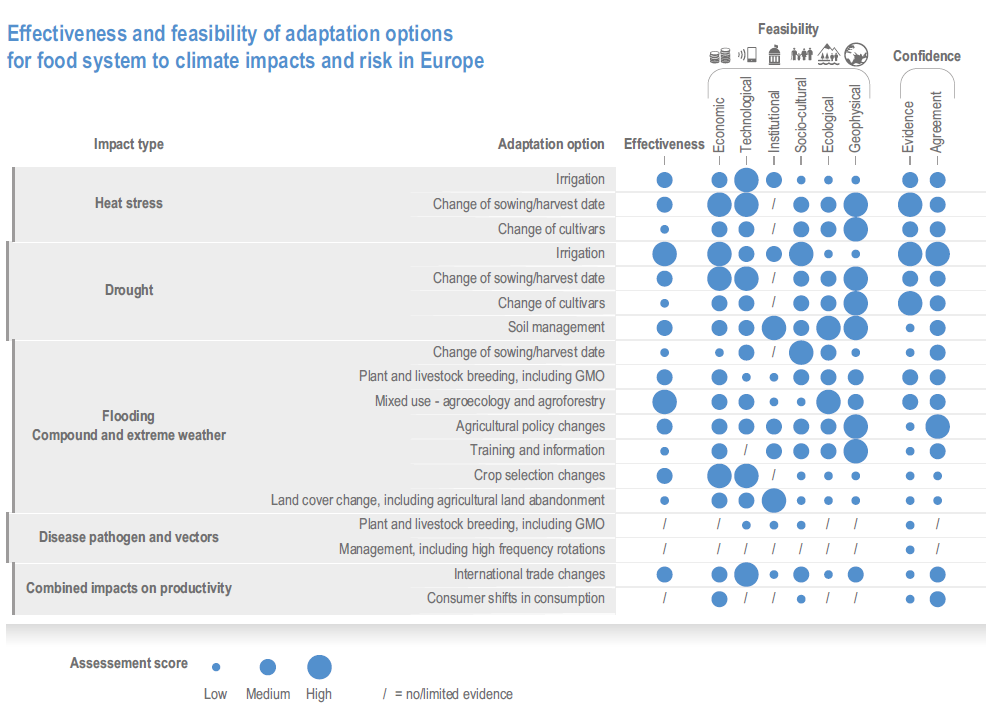
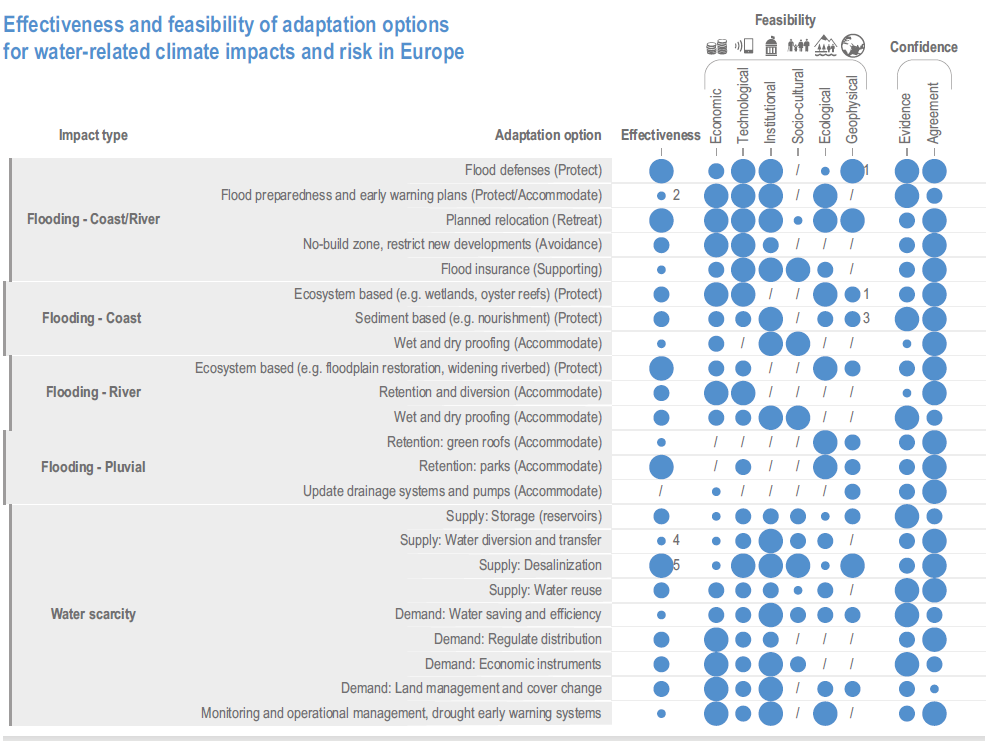
Figure 35. Feasibility and effectiveness of adaptation options for risks and impacts related to water and the food system. Source: IPCC, 2022.
Adaptation planning can be both supply- and demand-driven (Figure 36):
- Supply: seeking to increase the availability and supply of fresh water by storing water, diversifying sources and diverting and transferring water.
- Demand: monitoring (e.g. water meters, drought early warning systems) and regulation of demand, e.g. water restrictions, water pricing, or water savings and efficiency measures,
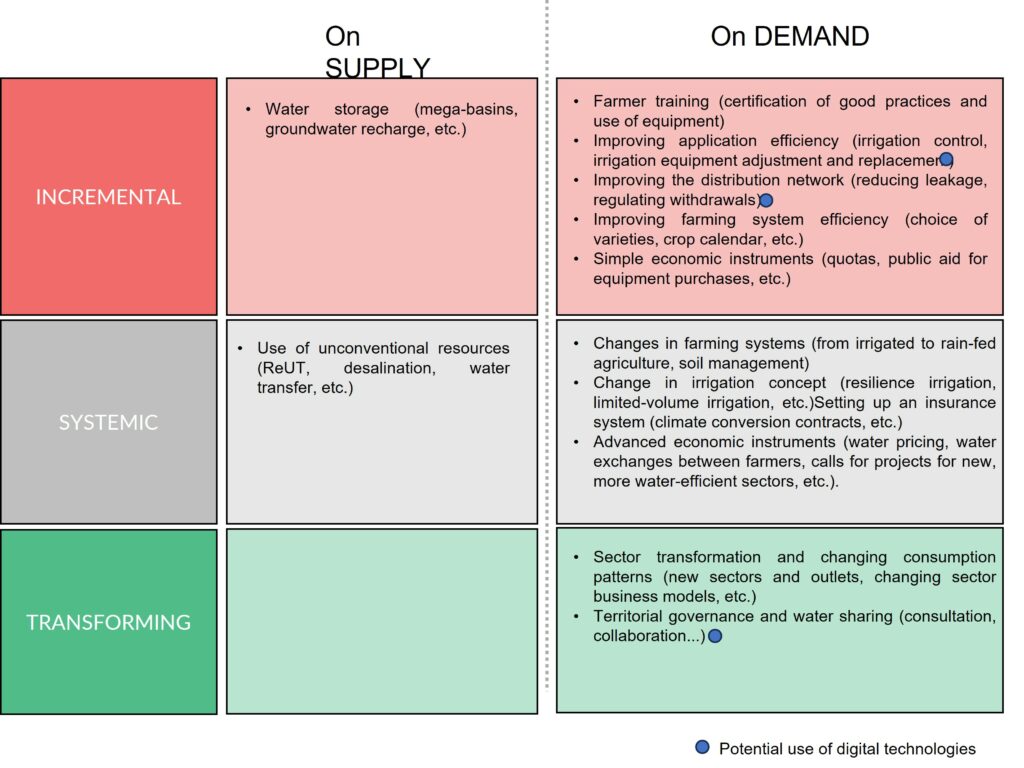
Figure 36. Summary of adaptation options based on supply and demand (with a particular focus on digital technologies in certain options). Source : the author
Adaptation is progressing, but remains insufficient in the face of rapid change. The IPCC reminds us that a coherent set of measures, including sobriety measures, can reduce risks, and confirms that the development of reserves and inter-basin transfers creates inequalities of access and perpetuates maladaptation practices. With warming of around 2°C, transformational measures become necessary. Around 3°C, even a very large number of coherent adaptation measures cannot guarantee to avoid water shortages.
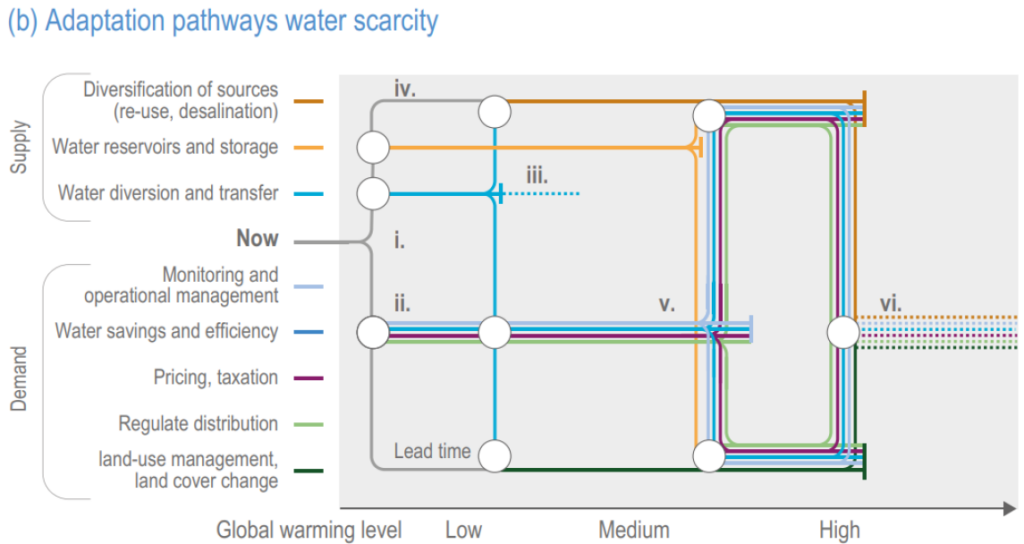
Figure 37: Accessibility of adaptation measures for water management in a rising climate. Source: IPCC, 2022. Note: (i) Currently, there is already a growing gap between water demand and water availability in some parts of Europe, (ii) A package of demand-side measures can reduce the risk to a medium global warming level, (iii) Water reservoirs and transfers can have distributive impacts and, when used for irrigation, intensify water dependency, (iv) Desalination is effective and can be developed, but has negative effects on the environment and energy demand. Water reuse is effective, but depends on water availability and the lead time for setting up infrastructure is long, (v) with medium global warming, demand-side measures need to be combined with transformative measures such as land-use changes, (vi) with high global warming, a broad combination of measures is needed to sufficiently reduce the risk of water shortages, and it may not be possible to avoid water shortages.
Adaptation must also be considered from a strategic point of view, in terms of what we want to see happen in the future. The first challenge is to ensure the viability and continued existence of sectors, some of which must be considered strategic for our country (e.g. the protein plan) or essential in terms of food self-sufficiency. Certain crops, for example, whose irrigation is imperative for the quality and regularity of supply to the agri-food factories linked to them, run the risk of being relocated.
Let’s not forget that the problem is global. If we reduce the amount of water we use at home, but virtually import it (which is what we’re doing now, given the deterioration in our trade balance), especially when this water comes from countries poorer in water resources than France and more vulnerable to climate change, we run the risk of bringing them down first (which is a problem in itself) and then us, since their export crops will no longer be guaranteed in the long term. Tunisia’s national water company, Sonede, for example, began cutting off the water supply every evening between 9 p.m. and 4 a.m., during Tunisia’s violent droughts.
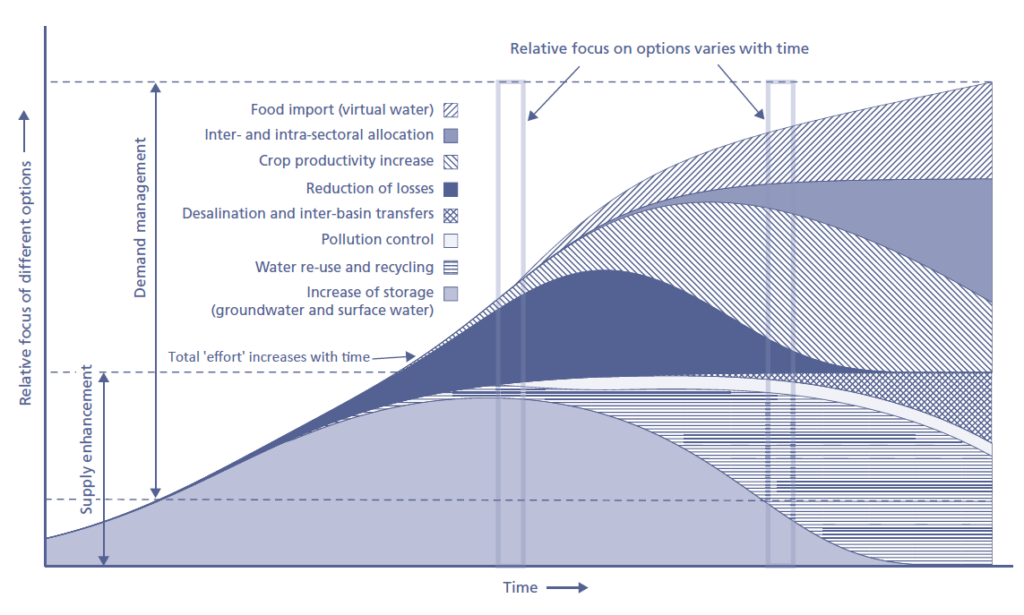
Figure 38. Relative importance given to different options for the agricultural sector to cope with increasing levels of water scarcity over time. Source: FAO (2012). Coping with water scarcity
The cost of adaptation
In 2022, the CGAAER highlighted the difficulty of making an exhaustive assessment of the costs of both impacts and adaptation in the agricultural sector, given the multi-faceted determinants of the production system and the close links between industry and territory. The aggregated assessment at national level (which does not provide information at finer spatial scales) of the adaptation of the agricultural sector amounts to 3 billion euros per year, with :
- one billion euros per year for climatic hazards, assuming a doubling of current hazards by 2050
- one billion euros per year for adaptation costs linked to increased use of irrigation at one billion euros per year (investment in new storage facilities and on-farm irrigation equipment, assuming a doubling of the volumes of water withdrawn for irrigation).
- 150 million euros to boost farm advisory services
- 600 million euros to renew France’s orchards, which are ill-adapted to the coming climate change.
It should also be remembered that in some French regions, following the withdrawal of ailing industries, plots of land benefiting from irrigation infrastructure have been massively urbanized, which has ultimately led to the loss of public investment in land irrigation, without any compensation having been put in place.
This cost of adaptation should be set against the cost of non-adaptation, which continues to rise exponentially as long as no action is taken. The cost of inaction is generally much higher than the cost of action.
Below is a list of studies that can be carried out to analyze the agro-climatic vulnerability of a farm and its adaptation to climate change.
Analysis of agro-climatic vulnerability:
- Description of crop rotation, with ranking of the farm’s crops in descending order of surface area.
- Review of the main climatic hazards and their agricultural impacts over the last ten years (crops, surface areas, level of yield losses suffered).
- Description of the farm’s agro-environmental characteristics: soil types, useful reserves, susceptibility to erosion, crop establishment practices, crop rotation and diversity, etc.
- Analysis of the farm’s current crops: surface area, genetic diversity, yield (minimum, maximum and potential variation) and main climatic factors reducing yield.
- Highlighting a selection of agro-climatic indicators chosen according to the farm’s main crops: comparative evolution between the Recent Past and the Near Future.
- Farm vulnerability matrix taking into account, for each crop, the frequency of hazards and the associated level of impact, making it possible to assign a current vulnerability score. The vulnerability score is then extrapolated to the Near Future by projecting the farming system as it would be in a changing climate.
- Study of the evolution of the organic state of agricultural soils according to current and projected cropping practices.
Adapting to climate change :
- Summary of the main climatic strengths and weaknesses of the agricultural system, as well as the climatic opportunities and threats to be anticipated for the near future
- Setting up an adaptation plan: vulnerability components and ranking of ESR (Efficiency, Substitution, Reconception) measures
- Evaluation of an adaptation plan with progressive dependence on irrigation.
Can we insure a collapsing world?
In all cases, should irrigation be considered as just another economic option (to be determined and managed by the agricultural sector) or could it, in certain configurations, correspond to an insurance necessity in the face of a climatic constraint imposed on farmers (legitimizing intervention by the community)? The IPCC points out, for example, the risk of loss of rural income due to insufficient access to irrigation water and reduced agricultural productivity, or the disruption of food systems as a result of rainfall variability or drought. Irrigation is a tool for both resilience and risk management. For certain crops, irrigation is both a yield and quality guarantee, as well as an anti-impact insurance policy. For example, if durum wheat is to be classified as semolina wheat, it needs to have a minimum protein content and sufficient nitrogen supply at the end of the cycle. In the context of a dry spring, the last nitrogen application will not be properly valorized, and we can therefore imagine that a passage of irrigation would ensure that the nitrogen is well assimilated.
A water-energy nexus
Will we run out of energy before we run out of water? The question may seem awkward or even provocative, but it’s a legitimate one in any case.
We are inundated with energy. Energy is so infused into our societies that we no longer even realize how ubiquitous it is. And in the context of water management, particularly in agriculture, this is particularly worrying. Take the case of a reel, for example, where over 80% of the electrical power is used to supply water (50% at the pump outlet and 30% at the reel inlet), with the remainder dedicated to irrigation. It is estimated, for example, that a corn farmer spends between €400 and €600/ha (including depreciation) on irrigation – a large part of the cost being energy-related. The explosion in energy costs following the war in Ukraine has certainly changed this situation.
The levers for energy savings are quite substantial. With regard to water distribution, modernizing water distribution networks would already enable savings to be made. Irrigation networks (pipes and other facilities) are the most significant element in life-cycle analyses, and these impacts may raise questions about the comparison of basic scenarios with hill reservoirs and/or pumping from the sea, versus a more energy-efficient scenario with a whole network to set up. As far as equipment is concerned, there are significant differences in energy consumption, simply between a reel and a pivot, for example. The Oscar irrigation robot (from Osiris) would visibly reduce pumping energy – the robot being powered by pressurized water.
It is to be expected that equipment will now seek to work at low pressure to limit energy costs. Some manufacturers supply drippers to operate at 0.5 bar nominal pressure. On pivots, we can operate machines with 1 bar of pressure at the head and sprinklers afterwards. When it comes to irrigation management as such, it’s easy to see that the greater the quantity of water saved, the greater the associated energy savings, since energy is needed to bring the water to the plot (water leaks are more of an energy problem than a water one, since the water potentially joins the small and large water cycles). And if we want better quality water, we’ll have to add filters of all kinds, the manufacture of which will have consumed energy. We could also add that remote valve activation systems (solenoid valve, pump…) reduce the energy used to travel to the control station.
Water is also a resource used directly for hydroelectric generation, which accounts for over 12% of France’s total electricity production. More than half of this production capacity is provided by so-called “run-of-river” facilities, which continuously turbine all or part of the flow of a river. They are therefore totally dependent on the available flow. Hydropeaking plants turbinate water stored for a short period of time in a river diversion, and are again highly dependent on the flow available in the river. Large-scale power plants backed by man-made reservoirs account for a quarter of total hydroelectric production. They are the most controllable, even if the level of reservoir filling limits production capacity. Finally, pumped storage power stations use water as an electrical storage device.
One issue that may arise is the renewal of hydroelectric concessions. With competition in energy production methods, concession renewal projects could be blocked at European level.
Water is also needed to cool nuclear power plants. The French Environment Code already provides for the possibility of modifying the standards governing water use by nuclear power plants to ensure continued operation in exceptional situations, such as a heatwave or drought. These derogations meet a general interest imperative: to continue to ensure the country’s electricity supply. I’d like to stress here that, in France, a shortage of water to cool power plants does not entail any nuclear safety risk (power plants are not going to explode if they can no longer draw water) – the risk is that they will no longer produce nuclear-generated electricity. Cooling water for the primary circuit must not be confused with that for the secondary circuit. These two circuits do not exchange any drops of water. The primary circuit is where the uranium rods are bathed. Water, in a closed circuit, serves as moderator and coolant. And indeed, if the water runs out, there’s no more moderator, and the fission chain reaction can physically no longer continue. However, this primary circuit has nothing to do with water taken from the river or the sea.
In the medium term, the sustainability of power plants that consume a lot of water and discharge water that is hotter than that taken in, i.e. open-circuit power plants, is questionable – particularly for those located on the coast (because for those located near the sea, it is assumed that the quantity of water will sufficiently dilute these heat effects). I would remind you that the proportion of open-circuit and closed-circuit reactors in the installed base in France is almost equal, and that open-circuit reactors consume the most water (even though they return almost all the water to the environment). According to EDF, it would be too difficult and costly to convert a unit cooled by an open circuit to a closed circuit. In addition to the RTE study on energy futures in 2050, published at the end of 2021, EDF is conducting a study (still in progress) whose preliminary conclusions suggest that the frequency of climatic unavailability of its nuclear power plants could be multiplied by 2 or 3, and would therefore remain low in relation to total energy production (the loss today being 0.27%). The profitability of the sites is therefore not called into question in the medium term. However, the French nuclear safety authority has reportedly asked EDF to anticipate droughts at these plants in the coming summers.
Drinking water supply is also subject to a significant energy infrastructure, with systems for pumping water from rivers and aquifers to reservoirs, for potabilizing water (e.g., chlorination to eliminate bacteria), and for purifying wastewater. Some of the water resource enhancement measures we briefly described at the start of this dossier are more or less energy-intensive (technologies for reusing treated wastewater, desalination or water transfer).
The expected power cuts, particularly in the context of load shedding alerts on the French electricity grid during the winter of 2022-2023, raise questions about our ability to maintain our water supplies. Some systems, such as potabilization and water purification, are not priority users and could be shut down in the event of a power cut.
In France, even if the risk of load shedding is real over the next few years, we are fortunate in that it is normally limited in scope. Nevertheless, in France, water management is a decentralized responsibility. This means that not all regions are on the same footing, and therefore not all are equally resilient in the face of an energy shortage. Planning and preparation remain essential, all the more so as climate deregulation raises the risk of increasingly frequent extreme events. In addition to the direct risks to energy supplies and agriculture, extreme events can weaken infrastructures, reducing the system’s ability to meet needs when they are most essential.
With less oil, perhaps the community will decide that there are more essential things to do with the oil we have left than to use disposable plastic bottles to transport a product that arrives just as easily through the pipe.
Water allocation mechanisms
There are several mechanisms for allocating water to local users (whether or not they are irrigating farmers)
- Public allocation of water, whereby the State decides on the quantity of water allocated to different uses. This is the principle behind water abstraction quotas, for example.
- Water markets, i.e. the trading of water rights;
- Pricing, i.e. the use of water is subject to a charge.
The term economic instruments covers a wide range of different tools: tariffs, taxes, subsidies, tradable permits [quotas]. Clearly, economic instruments have their limits. Setting up and applying these approaches often involves significant transaction costs. Economic instruments are also unlikely to achieve optimal resource allocation or pollution reduction if they are not designed with these objectives in mind.
Quotas
Quotas are a tool for managing irrigation water demand. They distribute the resource among users by limiting the quantity that can be withdrawn. They are nominative, geographically localized, capped and limited in time. However, quota systems exist in a variety of hydrographic and political contexts, under heterogeneous forms and denominations.
Quotas are often put forward with four frequently cited objectives:
- Equity: Quotas would ensure fair distribution, guaranteeing equal opportunities for irrigators to access the resource. They would be more effective than price regulation in reducing social inequalities. However, equity remains a vague concept whose definition depends on the context in which it is used.
- Economic efficiency: Quotas would enable us to maximize the marginal productivity of the resource. However, quotas represent a relatively rigid administrative constraint.
- sustainability: quotas limit the quantities that can be extracted for agricultural use and can therefore prevent overexploitation to limit pressure on the environment
- food self-sufficiency: Quotas are sometimes also introduced to guarantee a particular agricultural production (quantity or type of crop), or even to meet a country’s objectives in terms of food self-sufficiency.
Quota systems, over and above their common characteristics, fit in with local issues and constraints in a variety of ways.
- Allocation of quotas: Initial allocation of quotas means determining an allocation criterion and an indicator to express this criterion. Criteria can take a variety of forms, such as equity, seniority, the location of the extraction point, or the use of the resource.
- The integration of individual quotas into collective management: Quotas are allocated to specific economic units and are therefore individual, but they can also be managed collectively, for example with individual quotas resulting from the sharing of a collective quota, or individual quotas managed collectively. The volume of water that can be withdrawn by irrigated agriculture can therefore be assimilated to a collective quota, issued to an OUGC (in France, it is an unique organization for the collective management of irrigation withdrawals), which is then responsible for sharing this collective quota between irrigators, by determining individual quotas, which replace individual withdrawal authorizations.
- Adapting quotas to the unavailability of the resource: Quotas are a means of responding to the structural unavailability of the resource. However, this resource may be subject to a one-off shortage, which means that the quotas already defined need to be adapted, for example by prioritizing quota beneficiaries (according to seniority or crop type, for example), and/or by sharing the shortage proportionally between irrigators.
- Quota transferability: Quotas allocate a limited quantity of water to a specific user. Exchanging quotas makes the resource more flexible, with either non-transferable quotas, quotas transferred informally, quotas transferred only marginally and temporarily, or fully transferable quotas.
The quantitative sharing of the resource between users, achieved by quotas, presents a great diversity of implementations, allowing quota systems to adapt to local constraints. Different combinations of these characteristics can satisfy one or other of the objectives of quota systems. Quota systems in France are built on common features at national level, but adapted to local situations, making them different from one watershed (or sub-watershed) to another.
Surprisingly, the word quota is never used in the legislation. A volume that can be withdrawn is shared between irrigators in annual allocations after apportionment, replacing “individual abstraction authorizations”. The term quota is replaced by terms such as “authorized volume”, “withdrawable volume” and “water quota” to refer to the collective quota, and terms such as “approved volumes”, “revised authorizations” and “annual allocations” to refer to individual quotas.
Water pricing
Challenges to water pricing in agriculture include cost recovery issues, such as the generally high financial costs of setting up irrigation systems, as well as difficulties in controlling groundwater use and unauthorized water abstraction
The level of the levy seems perhaps too low for it to effectively act as an incentive for user behavior. And for a very long time, in the agricultural sector in France, there were no royalties on the quantities withdrawn by agriculture. Some irrigators are even exempt from the levy if they draw water below a certain threshold.
The vast majority of farmers still pay on a flat-rate basis. Large consumers are therefore not penalized. What’s more, this calculation system does not contribute (or not sufficiently) to the operating costs of the facilities. Some operators have introduced a more incentive-based and efficient pricing system, with a fixed portion calculated on the basis of surface area or subscribed volume, and a variable portion calculated on the basis of volume actually consumed.
Pricing is based on a whole host of assumptions that are not necessarily imagined at the outset. There are three main types of pricing applicable:
- Flat-rate pricing: linked to a subscribed surface area or, what amounts to the same thing, to a volume initially subscribed by the irrigator. While flat-rate pricing has the advantage of being very simple to implement, it has the major disadvantage of disconnecting cost recovery from actual water consumption. This type of pricing is mainly used for gravity-fed irrigation.
- Proportional pricing: invoicing is a direct function of the volume of water abstracted. Proportional pricing presents a risk for the waterworks owner-manager, since in wet years, when consumption is lower, it may be difficult to cover fixed costs. Proportional pricing can take a number of forms: constant, cross-level, seasonal, block, etc.
- Dual pricing: this comprises a fixed part, linked to a subscribed surface area or flow rate (or even volume), and a variable part, linked to the volume actually consumed. Several managers of collective irrigation networks (development companies) and ASAs use dual pricing, which seems to be the best way of establishing a fair match between actual irrigation use and contribution to costs.
Water efficiency initiatives have consequences for the economic model of water and wastewater services. Basically, if everyone started using very little water, with a low tariff for the first m3 used, managers would lose money. What pricing models could be implemented to disconnect part of the revenue from the volumes consumed? There don’t seem to be any miracle solutions. For example, some of the people I interviewed tried to propose tariffs that would penalize corn crops, thus enabling farmers with more diversified crops to irrigate throughout the season. Such pricing would have been widely rejected by the farming profession, in particular following pressure from influential corn growers in the region.
The financing of public action in favor of water is based on three strong principles on which there is a broad consensus:
- 1st principle: “water pays for water”. The financial resources collected from water users must be used to finance the investments needed to improve water management, and nothing else. This closed financial circuit translates into the collection of fees payable by users, and not taxes spread across all taxpayers that would be unrelated to their degree of water use.
- 2nd principle: “polluter pays”. Those responsible for damage to water resources must pay for measures to restore them to good condition. The principle is simple, but its implementation depends on the ability to quantify environmental damage and identify its sources.
- 3rd principle: “upstream-downstream solidarity”. This financial solidarity is implemented on the scale of the hydrographic district, i.e. on the perimeter of each Water Agency.
The simplicity of these principles does not preclude a certain complexity in the financing of water policy, which involves a multitude of players with sometimes highly disparate financial capacities, whose water management infrastructures are, for historical reasons, at highly variable levels, as are their needs and projects.
Water users’ bills cover the cost of the service rendered, but also include a proportion of fees intended to finance the water policy pursued by the Water Agencies, which constitute their main resources. The communal block has been endowed with a dedicated resource through the GEMAPI tax (in France, management of aquatic environments and flood prevention), which is an optional local tax added to the property tax.
While most of the financial resources of the Water Agencies are taken from domestic water users, the proportion of funding returning to the small water cycle through subsidies to water and wastewater services has been steadily falling. To add insult to injury, the Water Agencies are penalized by the system of the biting ceiling. They do not retain all their revenues. Their expenditure is also capped, with special financial controls. As the Agencies’ subsidies are reduced, the self-financing provided by intercommunal bodies has had to increase. Unwilling to increase water charges, local authorities have had to find the resources themselves to continue their investment programs. With the downward trend in water consumption, volumes billed are declining, and only an increase in the rate of water charges would enable water and wastewater services to maintain the same level of revenue.
Water market – Water privatization
The most direct way to appropriate a resource is to privatize the resource itself, i.e. to allow its purchase by a private company. Privatization is thus accompanied by the phenomenon of commodification, as water becomes a commodity that can be appropriated according to the costs set by the market. By financializing it, the value of the resource is distorted, since it is disconnected from this very material reality. In concrete terms, the financialization of water involves several mechanisms. On the one hand, individuals can invest in the companies that operate and manage drinking water through the purchase of shares (in Véolia, for example). On the other hand, it is now possible to bet, through the purchase of financial derivatives, on the eventual price of water, based on changes in quantity and accessibility.
With the increasing scarcity of water resources, the idea of commoditizing water resources has gradually gained ground, and some countries (USA, Chile, India, etc.) are already experimenting with local water markets, with varying degrees of success. At the same time, many voices are being heard at international level to prevent such a process from becoming widespread, arguing that water is a common heritage of humanity.
Advocates of water markets often forget to specify what is meant by a water market (which takes many different forms, such as the exchange of canal water towers between farmers, the transfer of water rights from the agricultural sector to the urban sector, or the reallocation of water resources between different countries), and equate the water market with the ideal functioning of markets based on pure and perfect competition. In theory, however, a market based on pure and perfect competition must meet five conditions to achieve efficiency: atomicity of supply and demand, homogeneity of the good exchanged, market transparency or perfect information, fluidity of demand and mobility of supply. And it’s relatively easy to show that most of these conditions are not met in a water market. It should also be remembered that many water markets are highly administered. Water markets are not necessarily synonymous with privatization, since the state may grant user rights but retain ownership of the water.
In 2003, Australia launched the world’s first water buying and selling exchange – Water Find. On the Australian market, this exchange acts as a mediator between consumers and the market. As the overwhelming majority of water consumption is linked to agriculture, it is this sector that is primarily targeted for privatization. Irrigation water quotas are allocated to farmers, who can then trade their surplus on the market.
In Spain, the volumes of water traded on these water markets (trading centers – also known as water banks – and transfer contracts) have always represented a tiny fraction of the total volume of water allocated and used each year, even in times of drought. Not only have these markets failed to make water sharing more efficient and sustainable, they have also exacerbated the dysfunctional nature of these markets, with concessions concentrated in the hands of large, intensive farms producing high-value, water-hungry crops, under-allocation of water for aquatic environments, and excessive pumping by farmers from ground and surface water.
On December 7, 2020, NASDAQ (Wall Street) and CME (Chicago Stock Exchange) announced the opening of a water futures market in California. The index used, NQH20, represented the average price of water based on volume, without taking into account water losses and other costs. The index varies according to weather conditions. With just a few clicks, anyone can buy shares potentially equivalent to several thousand cubic meters of water, and hope that the next drought will drive up prices.
Symbolism aside, by 2023 this futures Californian market looks set to be a failure, with transaction volumes ultimately very low. This type of market, described as illiquid (because there aren’t enough transactions), has several limitations:
- Different regulations apply to surface water, groundwater and water from treatment processes
- Water scarcity varies greatly (spatio-temporal water heterogeneity) between hydrological and administrative basins.
- No water deliverable at the end of the contract in a context of drought and water shortage
The lack of information and transparency on physical markets discourages speculators and other investors from entering the futures market. More importantly, no other water futures market has appeared since, even though most opponents of the financialization of water feared the multiplication of such markets across all financial markets.
What undoubtedly saves water from total privatization is the perception of its abundance. Over 70% of the earth’s surface is occupied by bodies of water, but freshwater accounts for only 3% of the total (see dossier introduction). In addition to this, it should be remembered that a market works well when there is a stock to manage. To manage a flow, it’s much more complicated (the markets in place in Chile and Australia are there to manage stocks). A water market also requires that constraints be kept to a minimum (for example, spatial constraints on water exchange should not be imposed), at the risk of cancelling out all the benefits linked to the market, which would be unable to allocate water where it is best used.
In the case of water resources, however, there may be important reasons why market allocation is either undesirable or impractical. These difficulties arise in part from the fundamental importance of water to the maintenance of biological, social and economic systems. Ecosystems need water to function properly and provide services. Human beings need water to survive and prosper. Simply allocating water to the highest bidder is potentially highly problematic if humans or ecosystems are unable to meet their basic needs.
The omnipresence of water, its mobility via the water cycle and the enormous diversity of uses for water make the task of creating markets a difficult one (we might even mention the air basins mentioned at the start of this dossier). Water is unusual in that, depending on context and use, it can have the characteristics of a public good, a private good or a common good. In the absence of fully functioning markets, water prices fail to reflect the environmental impacts of water use and the opportunity costs (i.e., the maximum value that could have been generated by using water for other purposes). The result is market failure: misallocation of resources and a sub-optimal balance between economic activity and the pollution it produces.
However, this failure should not overshadow the increasing financialization of water. In addition to this financialization, other mechanisms such as “cap and trade” markets operating on the principle of a maximum ceiling (cap) and exchanges of allocated quantities (trade) are conceivable. This is already the case for the carbon market, and partly true for the water market, where quota systems and local management or trading have long been in place. These exchanges of water rights can be permanent or temporary.
In France, water markets do not exist. The main limitation is legislative. As water is considered a national common heritage, it is not a monetizable resource as such. Water markets are also ill-suited to individual pumping installations, which are in the majority in France.
Nevertheless, we could see the emergence of local water markets in times of crisis – in the form of quota management by OUGCs (in France, single bodies for the collective management of irrigation abstractions), which could allocate water to farmers who make the best use of it. Irrespective of the crisis, the OUGC could resemble a water market manager insofar as it centralizes agricultural requests and decides to allocate water to farmers according to availability and existing authorizations. The OUGC could define operating rules that could resemble those of a market (for example, by allocating more water to those who make the best use of it). By favoring the allocation of water to high-value crops, we are in fact witnessing a derogation that is a form of water market based on the economic valuation of water.
Pseudo water markets can also be observed from time to time, when farmers hold on to their abstraction authorizations only to sell them on to others in critical years. Which, incidentally, completely cancels out the effect of a water restriction policy.
We could also imagine arboriculturists being granted an irrigation dispensation and, by avoiding losing a large part of their production thanks to irrigation, compensating hay producers for the loss of production suffered that year, who would not have had the right to irrigate (we talked about this in an example on the Crau plain). Monetizing water remains a problem for many. The concept clashes with reasoning, despite the fact that some farmers stand to gain from it.
Why take the risk of leaving water management to the market when we have the regulatory instruments to do so? Water is privatized from the moment it is used, except that authorization is required for its use. This authorization is regulatory and is not due to the propensity to pay more than one’s neighbor.
Water speculation is perhaps even more insidious than the markets we’ve been talking about. In France, there is no speculation on the price of water, but on access to water. The notion of price is not apparent. In reality, we speculate on the future value of water. Some people want their land to be irrigable for short-term insurance reasons, but also for speculative reasons. Land with access to irrigation water generally sells for more than land without access to water. Unlike carbon, water is localized in space. Unless you have very heavy infrastructures to move it around, water management is totally different from that of carbon, which is potentially exchangeable everywhere.
For example, there is a strong demand for vine irrigation in the Occitanie region. Is it the role of the state or the regions to subsidize such infrastructure for speculation, knowing that in the end, some will have access to water and others won’t (on the assumption that not everyone will be served), all from public funds? What priorities should be set, given that certain food crops could, on the contrary, really benefit from surplus water? At the present time, and given the price of energy, it’s better for some people to have a significant economic value behind agricultural production.
The privatization of water is also hidden behind the growing hold of multinational water (Véolia), water infrastructure (Vinci, Eiffage), hydroelectric and nuclear companies on the huge public contracts that droughts, storms and floods create. In January 2022, the French multinational Veolia became the 86.22% owner of its main competitor, Suez, on which it had launched a takeover bid a year earlier. This operation gave Veolia a near-monopolistic position in the water management market.
The process is somewhat similar with bottled water. With three major companies dominating the sector – Neptune, Nestlé Water and Danone – the aim is to buy, treat and package municipal water, which is sold at very low prices, in order to resell bottled water at much higher prices.
How do we manage water collectively?
Reconsidering water governance
Water is part of the nation’s common heritage. This is enshrined in Article L 120-1 of the French Environment Code. But we forget that water is first and foremost a local good, with contexts that vary enormously from one territory to another.
A brief legislative history of water in France: what does it look like?
- 1964 (French law). Water agencies were created to collect water charges and finance projects to preserve and restore water resources to a good state. The “polluter pays” principle was introduced. The Agences de l’Eau (known as Office de l’Eau in the French overseas territories) are public administrative establishments of the French State, each operating within the boundaries of a hydrographic basin. Water is therefore managed on the basis of large watersheds.
- 1992 (French law). The law establishes water development and management master plans (SDAGE) for each of the 12 major river basins in metropolitan and overseas France, and their local implementation through water development and management schemes (SAGE).
- 2000 (European Water Framework Directive – WFD) It sets 4 objectives: non-degradation of resources and environments, good status of water bodies, reduction of pollution, compliance with standards in protected areas. The original deadline of 2015 has been pushed back to 2027. National implementation of measures to improve the status of water bodies has fallen behind schedule, and the target of 100% of water bodies complying with standards by 2027 already seems difficult to achieve.
- 2006 (French Law – LEMA): The Law on water and aquatic environments incorporates the WFD and takes climate change into account. It overhauls the principles of water pricing. For basins where deficits are particularly linked to agricultural abstractions, a provision allows for the creation of unique organizations for the collective management of irrigation abstractions (OUGC), responsible for defining the criteria for the distribution between irrigators of the withdrawable volumes set by the administration. The volume that can be withdrawn for irrigation is allocated by the prefect to the single organization, which is responsible for apportioning this volume among all irrigators each year.
- 2014 (French law – MAPTAM) and 2015 (French law – NOTRe). These laws give GEMAPI (gestion des milieux aquatiques et la prévention des inondations – management of aquatic environments and flood prevention) powers to EPCIs (établissements publics de coopération intercommunale – public establishments for inter-communal cooperation), reflecting the transfer of water and wastewater management powers from local authorities to inter-communal bodies. While two-thirds of drinking water services and three-quarters of wastewater services are managed by local authorities, delegated and managed services are more or less equal in terms of the number of inhabitants served. The MAPTAM law created the status of Etablissements publics d’aménagement et de gestion des eaux (EPAGE – public water development and management establishments) for syndicates operating on the scale of sub-basins or coastal basins.
- 2019 Ministerial instruction: arrival of PTGE water management projects (more on this later).
- 2023 National Water Plan launched by the government
All these laws have profoundly altered the institutional landscape of the water sector. For the time being, most local authorities are refocusing on their compulsory competencies (Gemapian authorities – in reference to GEMAPI competency). Quantitative water management remains somewhat of an orphan, with the exception of water agencies. This observation can be paralleled by the flourishing of individual or pseudo-individual (GAEC) micro-projects, for exclusive agricultural use, routinely sized to fly under the radar of water regulations. Nevertheless, there have been some interesting initiatives, with the creation of mixed departmental or interdepartmental syndicates that bring together one or more departmental councils and local authorities (agglomerations, communes, EPCIs, AEP or sanitation syndicates), or even ASAs (authorized syndicated association).
In a report published in 2022, the French National Audit Office (Cour des Comptes) considers that water policy is both devolved and decentralized. Decentralization is said to be incomplete, entrusting significant responsibilities to local authorities, combined with ongoing State intervention that lacks coherence. The three competent ministries (environment, agriculture and health) are said to be defending different orientations, the differences between which have never really been overcome. The French Cour des Compte points out that the current organization is ill-suited to the challenges of quantitative water management.
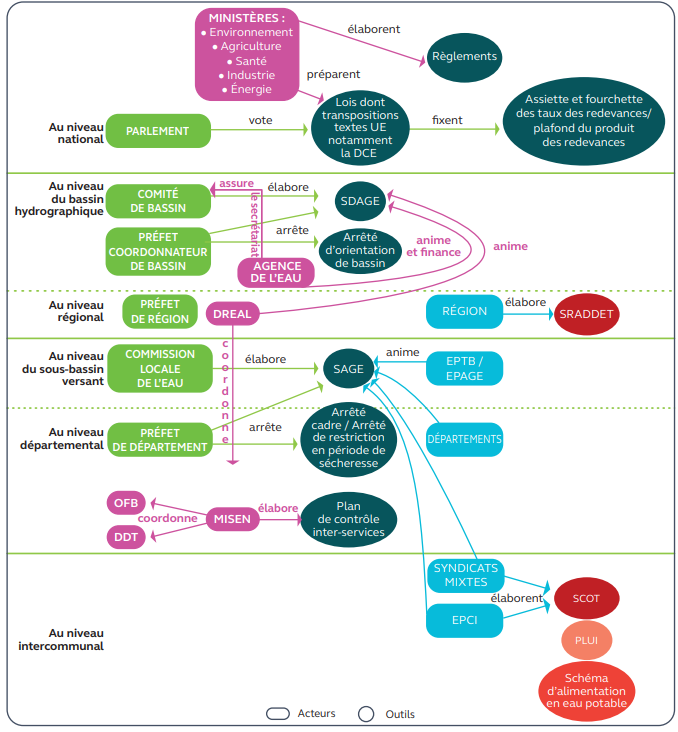
Figure 39. Simplified diagram of water governance in France. Not all players are represented…
Intervention by local authorities suffers from fragmentation, and is too often carried out on an inappropriate geographical scale. The mismatch between administrative boundaries and the geography of river basins and sub-basins is an inescapable reality, forcing the State and local authorities to set up numerous coordination bodies.
Transferring responsibility for managing aquatic environments and flood prevention (Gemapi) to public establishments for intercommunal cooperation (EPCI) would not have improved the situation. What’s more, as the territory of EPCIs does not correspond to that of hydrographic sub-basins either, the creation of public water development and management establishments (Epage) or territorial public basin establishments (EPTB) is necessary to ensure that a coherent policy can be implemented at the right scale, that of hydrographic sub-basins.
The French government has sought to integrate the specific nature of water management into its organization, by setting up basin coordinating prefects, who play a role in coordinating and ensuring the coherence of government action between departmental prefects, on the scale of large basins (those of the water agencies). Small watersheds, on the other hand, have concerted management mechanisms, such as SAGEs or PTGEs (we’ll talk about these shortly), which are well suited to these human-scale territories. On the other hand, the management, particularly quantitative management, of intermediate-sized sub-basins (large tributaries of our rivers, which cross several départements or regions) often poses problems, due to the lack of tools adapted to this scale. Today, it is essential to ensure that overall water management is planned and organized on an operational hydrographic scale throughout France.
Water policing powers are exercised by government departments, in particular the DDT (Departmental Territorial Directorates), but also by agents of the OFB (French Biodiversity Office). Prefects can call on the services of the DDTs, which are also responsible for the sea (DDTM) in coastal departments, and which examine authorization applications under the Water Act and promote local policy in favor of water and aquatic environments. They can also rely on the DREALs, which ensure that water-related legislation is properly applied, monitor classified installations and track river flows.
Schémas directeurs d’aménagement et de gestion de l’eau (SDAGE – water development and management master plans) are water policy planning tools for each river basin (seven in mainland France and five overseas). They integrate agriculture mainly in a prescriptive form, through the levels of water quality and aquatic environments to be achieved or restored, often using regulatory tools: reduction of nitrate or phytosanitary pollution, protection of drinking water supply catchments in relation to human health; preservation/restoration of aquatic environments (ZH, emptying of reservoirs, reserved flows), low-water target flow (DOE) and water savings on the quantitative side. Basin committees have also drawn up plans for adaptation to climate change, but these remain on a very broad scale and are difficult to implement at local level, where they are still little known and little used.
SDAGEs also have an effect on local land-use policies, since Schémas de cohérence territoriale (SCoT), local urban plans (PLU and PLUI) and communal maps must be compatible with SDAGE objectives. The same applies to the schéma régional d’aménagement, de développement durable et d’égalité des territoires (SRADDET – regional planning, sustainable development and territorial equality scheme), which must take balanced water resource management guidelines into account. Despite the fact that water development schemes and regional planning documents are linked, the notion of compatibility remains rather broad and vague. It is mainly based on the principle of non-contradiction, which is understood in different ways depending on the territory. It has to be said that the relationship between water and agriculture is often ignored in SCoTs and PLUi, that many PCAETs remain at a descriptive stage, and that the SRADDETs led by the regions are unevenly ambitious and often fail to include choices or options with regard to possible tensions between water and agriculture. Moreover, the French Environmental Authority highlights the risk of contradiction in the decision not to deal with this issue in its own right, when several provisions of the SRADDET, concerning other themes, could increase conflicts of use, particularly in sectors with a proven quantitative deficit. SDAGEs can thus act as a brake on urbanization when water resources are insufficient or degraded.
In these large-scale planning documents, the question of irrigated perimeters remains largely unaddressed. Irrigation facilities are not considered in the identification of agricultural land to be protected. Once the best lands have been identified, those with irrigation infrastructures will be considered for protection, with their potential irrigation reasoned in accordance with the other uses of the resource (drinking water supply and good environmental status), and the economically viable agricultural project planned.
The subject of irrigation does, however, raise its share of questions: How can we define the irrigable perimeters to be preserved in a context of reduced water availability in the future (basically, how can we define the irrigation terminals that could be maintained in a context of constraints) and where the sectors and crops present on these perimeters may evolve with climate change? How can we compensate for the loss of productivity linked to the loss of irrigated areas? How can agricultural planning (still in its infancy) and urban planning be better reconciled? And, more generally, how can we move agriculture away from a single-sector approach to develop a territorial approach, including water resources? What are the compensation mechanisms for the artificialization of irrigated land? What observatory should be set up to monitor the price of irrigated and non-irrigated land? How can we characterize inequalities between farmers who benefit from irrigation infrastructures and those who don’t?
The first difficulty is to know what is meant by “irrigable” land, on a national scale or more specifically for an operational land planning perimeter (SCoT, PLUi, PLU). The definitions of the European Union, the agricultural census and other local definitions are not always consistent with each other. Some areas have in fact been shaped by major hydraulic developments, sometimes dating back thousands of years, which closely associate water and agriculture. However, there is a certain vagueness, or even ignorance, linked to the fact that the surface area of irrigated land varies according to the climatic context at any given time, and that the definition of the term “irrigable” is subject to a variety of definitions, with little up-to-date data available at national level.
While the SDAGEs plan water policy at the level of each river basin, detailed management of water resources cannot be carried out at this scale. The SAGEs implement the SDAGE guidelines on a basin-by-basin basis, in a spirit of compatibility. SAGEs, which are sometimes criticized for being cumbersome and lacking coordination with other territorial planning tools, are not the only tools for local planning and regulation of water resource management. Environment contracts (river, lake or groundwater contracts), which are more flexible and concrete, can be signed between partners (State, Water Agencies, local authorities), under the aegis of an umbrella structure, to improve water quality or better manage available quantities.
Nearly half of all sub-watersheds are not covered by a water development and management scheme (SAGE), even though their preparation is a prerequisite for the practical implementation of SDAGE guidelines. Where they do exist, the content of these schemes is not always satisfactory, due to the average time taken to draw them up (close to ten years), the age of the data on which they are based, and the absence of targets for reducing water consumption.
In all basins where there is a quantitative deficit, prefects have been asked to determine the volume that can be withdrawn, for all uses combined, to guarantee the proper functioning of the corresponding aquatic environments and thus compliance with the low-water target flow (DOE) eight years out of ten, and to revise abstraction authorizations so that the total volume authorized is at most equal to the volume that can be withdrawn. For basins where deficits are particularly linked to agricultural abstractions, a provision of the 2006 LEMA law enables the creation of unique organizations for the collective management of irrigation abstractions (OUGC). For water abstractions intended for agricultural irrigation, farmers can now organize themselves collectively within unique collective management organizations (OUGC). These organizations hold a single, multi-year authorization to draw water for all their members, and annually allocate volumes between irrigators, implement any temporary restrictions, and organize water turns. As the volume that can be withdrawn is limited, new requests are put on hold until an irrigator is removed from the list. In the longer term, the main challenge will be to reconcile a diminishing supply of water during low-water periods (which are tending to last longer) with a demand that in some places is not being met, and will increase further as a result of climate change.
Irrigation developed through the development of collective irrigation networks and canal networks. From 1865 onwards, the managers of these collective networks gradually took the form of authorized syndical associations (ASA). ASAs are collective irrigation networks (there’s nothing associative about them). They are highly structured public establishments with the same role as a commune. At the end of the day, they are just another type of water user, and are customers of the OUGC. ASAs account for half of all collective water abstractions in France, the remainder being individual abstractions. ASAs are not involved in public water management policy, except for pricing within their own networks. Irrigation water is a charge like any other for ASAs. Spontaneously, the only reason why an ASA (authorized syndical associations) would set a high or dissuasive tariff would be to avoid exceeding their abstraction authorization, or to avoid over-investment in their network (need to set up booster stations, resize stations in certain places).
ASAs have to balance their budgets, so they have to juggle a number of objectives that limit their room for maneuver (balancing budgets, encouraging water saving, saving water and giving young farmers access to water, encouraging livestock farming to secure fodder, and encouraging the use of field crops in favor of livestock farming). The other period of significant development in collective irrigation began in the 1960s with the creation of regional development companies (SAR). Although it is difficult to estimate their relative importance, ASAs are thought to account for half of the irrigation carried out using collective networks, SARs for around 20%, and the remainder comprising a heterogeneous range of structures (communal networks, inter-communal syndicates, associations under the 1901 law, etc.).
The French state no longer has any engineering skills within its departments, and so has no competence to pass judgment on water management. For example, it is mainly the chambers of agriculture that manage the OUGC. The agricultural profession accepts the notion of water management (particularly sobriety) if it is accompanied by the creation of water resources. The chamber of agriculture, in its role as a departmental player, works on a scale that often does not coincide with the project territory. It is therefore not the bearer of SDAGE decisions, nor of the logic of the watershed or project territory. This may, for example, lead it to stick to positions of principle, or come into conflict with a neighboring chamber. Potential conflicts of interest may also arise, since the chamber is both a representative of agricultural interests and an organization that advises farmers or provides territorial expertise.
The PTGE (projet de territoire pour la gestion de l’eau, or regional water management projects) have been proposed as a means of managing water on a river basin scale in an operational manner. They follow on from the PGRE (water resource management plan) to encourage a process involving all water stakeholders in order to reduce the quantitative deficits observed in the territories. They incorporate a forward-looking vision designed to integrate the consequences of climate change on the availability of water resources, combining water supply and demand, and enable the opening up of the essential local dialogue on water sharing between agriculture and the other players in society, notably nature conservationists. The level of progress made by the PTGEs varies widely across France. They must be built in coherence with SDAGEs and SAGEs where they exist.
One of the French government’s proposals in response to the growing and sometimes virulent opposition from some sections of the population to the construction of water storage or transfer facilities for irrigation, is to draw up a territorial water management project (PTGE). It’s an endogenous approach to water management that aims to take into account the environmental, economic and social dimensions of water management, without dissociating them. It relies on collective intelligence to identify a trajectory for balanced management of the resource, and then to concretize it through a program of actions.
In practical terms, a PTGE applies to a catchment area (100 to 1,000 km2), often characterized by a specific problem (water shortage, flooding, water quality) to be reconciled with various economic activities (production, processing, tourism). The PTGE relies on existing, legitimate water governance structures, including the Commissions locales de l’eau (CLE), a kind of water parliament that draws up the SAGE and brings together all stakeholders to establish the project’s legitimacy (including, in particular, a Public establishment for water development and management (Épage) when they exist in the territory).
The PTGE tool seems well-suited to human-sized watersheds (enabling governance, co-construction and territorial dialogue between local players), but less relevant for very large watersheds, where it is important to anticipate the effects of climate change and organize upstream-downstream solidarity. Some local authorities are not sufficiently involved in coordinating PTGEs. The same is often true of the agricultural sector, which should be more actively involved, in particular to help bring about multi-stakeholder commitments in water and agriculture to make the implementation of the PTGEs operational.
Few PTGEs have yet been economically evaluated. Economic evaluation is important because it indicates whether the scenario studied is economically desirable and profitable from the point of view of each category of stakeholder. But it does not say whether this scenario is the best for water resource management, or for other environmental criteria that also need to be taken into account. The value of a scenario without a project is poorly understood, and without this reference situation, negotiation becomes the main tool for determining the trajectory to be followed. Cost-benefit analysis is the poor relation of the approach, even though it is a decision-making tool for the implementation of actions, particularly those requiring public subsidies.
Water policy is thus facing a crisis of legitimacy. Preserving balanced water management can only be achieved by re-politicizing the water issue, by asserting that it remains possible to better mobilize water for our needs without degrading the resource either quantitatively or qualitatively. The success of this strategy will certainly depend on strengthening the role of local elected representatives in the governance of water policies. The Local Water Commissions (CLE), which play the role of a local water parliament, are a body that would certainly benefit from being better mobilized.
The intertwined responsibilities of the State and local authorities are detrimental to the effectiveness of water management policy. Nor does it make it easy for citizens to understand the division of roles between decision-makers. More effective decentralization of powers would reinforce the responsibility of the various players in the management of this essential public policy, and make it easier for the general public to understand.
The problem is no longer one of having sufficient data. Historical meteorological data is available. Soil types are known locally. Climate projections, albeit fraught with uncertainty, still give a good idea of what could happen. Barring a completely unexpected and unforeseeable event, the trajectory is generally known. Collectively, we need to ask ourselves whether or not we are capable of sitting down around a table, listening to each other, and agreeing that together we will make choices based on data that will in any case not be 100% reliable.
A final question: in order to better manage water in France in the future, will a new law be needed to follow on from the 1992 and 2006 water laws? A number of water policy players have argued in favor of such a move, notably during a debate organized by the Cercle français de l’eau in the spring of 2022.
The need for consultation and collaboration on water issues
Conflicts of use, a recurrent term in water-related debates, usually reflect the difficulty of reconciling environmental and socio-economic issues. Each stakeholder challenges the legitimacy of the other’s use, with a different notion of willingness to pay and a fairly variable temporality of use between users. Water thus appears as a mirror of society. Elsewhere, it can reveal how society functions, but also mediate social relations. Through water, individual or group statuses are played out, as well as the reminder of the social order.
Even when water management is presented as autonomous and community-based, the influence of power relations between state and community can still be seen in the way the water’s path (choice of canal layout, location of sluice gates…) has been shaped, or even politically manipulated, for centuries. Why does water flow through a series of parallel canals in one place, rather than through a single, larger one? Why do canals cross each other in one place without their waters mixing, while further downstream they do? Why do so many different water-sharing techniques coexist in the same region? Today, with the development of boreholes, groundwater is at the heart of economic and political power issues. Collective irrigation networks have been remodeled (canals destroyed), in favor of individual groundwater irrigation.
Irrigation is too often seen as a purely technical or economic activity, and water as a mere resource. Yet a number of studies show that its management is above all relational, dependent on interactions between individuals, since activities such as water distribution and sharing, conflict management and infrastructure maintenance require collective organization and, at the very least, information. Water is also an element that links many areas of social life, and through it, social status, prestige, kinship relations and power relationships are expressed.
The question of irrigation requires a balance between the collective and individual dimensions. Self-management by communities of users is all the rarer to observe, as very often when farmers take the step, for example, of switching from surface water to groundwater, it’s to avoid all the constraints of the collective (we saw earlier in this dossier the tendency to disconnect from the collective network). By taking full individual charge of resources, there is no longer any sharing of water.
Irrigation can be seen as a form of insurance. An expenditure of money (reel, pumping water) provides a certain level of guaranteed yield. This insurance is managed on an individual scale, in the sense that each person takes a little of what he or she needs. However, this individual coverage could be pooled among several irrigators to manage risk in a concerted, collective way.
Water management often raises issues of trade-off (between efficient use, sparing use, or stopping irrigation altogether), as well as socio-economic issues. And these issues will become even more pressing as climate change reduces water availability and low-water levels are expected to fall. Why can agri-food groups pump spring water and sell it for several euros a liter when it belongs to everyone? Why must golf course grass be watered with drinking water? Do we want to deprive a currently water-efficient structure of water or one that doesn’t need it now but will need it in the future? In crisis situations, the real question is to think about an acceptable choice for optimizing the use of the resource.
What is fair? What is the least economically damaging? In agriculture, we might think that we should first secure irrigated crops that have no agronomic alternative in their edaphic and climatic context, strategic crops and those on which the balance of a local processing industry depends. But does this call into question the dominant agricultural model? And what about possible competition between energy crops (methanization) and food crops for access to water? The OUGCs (Organismes Uniques de Gestion Collective – single collective management bodies) are responsible for defining the criteria for allocating the prelevable volumes set by the authorities to irrigators. However, the rules for allocating irrigation quotas in terms of volume or flow never take into account priority for the most intensive crops in terms of value/unit of irrigation water consumed. Nor are there any collective pricing schemes to this effect. Perhaps it would be better to give priority access to those who have used as little water as possible in agriculture, as a sort of reward rather than a punishment.
Is there a possibility of solidarity between sectors in the event of a shortage of water? Basically, the question is whether some people are prepared to give up a little water for the benefit of others. Experiments were carried out on the Crau plain, in the Camargue region, to see whether it would be conceivable to reduce the allocation of water to hay producers and leave it to arboriculturists who, if water were to run out, would have much more to lose than hay producers. The aim was to see what price (level of compensation paid by arboriculturists, for example) hay growers were prepared to pay to agree to reduce their water use.
Unlike the notion of general interest, which is often abstract, social utility always refers to a specific intention. There is no such thing as social utility “in itself”. There is only social utility around an object or a project that a community of stakeholders shares, and which must be preserved, enhanced and developed. Identifying the social and territorial utility of a water and aquatic environment management project therefore always comes down to considering the site, environment and/or resource concerned as common assets shared by a group of stakeholders. In some cases, the aim may be to reinforce this common-good character, for example by extending the social appropriation of a site that has hitherto been little visited or reserved for a few initiates. Such an intention gives the project a political dimension which, while it may give rise to a few tensions or conflicts, is likely to facilitate its political support and the involvement of a wider circle of players.
Although a priori unifying, the question of the commons, and above all that of the limits of the community of actors who can enjoy it, can sometimes give rise to heated debate or even reticence, particularly when the places concerned have long been appropriated by a particular category of actors. The common good is not a fixed attribute: it is a dynamic process embodied and defended by collective political will.
Nevertheless, the issue of water has never been widely debated with the general public.
The sharing of water cannot be considered without identifying and widening the circle of stakeholders interested or potentially interested in the purpose of the project for water and aquatic environments. It won’t be enough simply to get farmers around the table. It also means providing the means to collectively build common ground within this community of stakeholders, notably through collective learning about the uses and issues at stake for each. Spaces for dialogue and exchange need to be created to bridge the gap of mutual misunderstanding between all players. Tools such as serious games (which may or may not be based on digital technologies) can help people to think collectively. This is the case, for example, with the RSEeau serious game, whose objective was to assess how to encourage farmers to aim for a slightly lower yield to smooth out peak demand, by means of quid pro quos (water rights at other times, money to compensate for loss of earnings, etc.). Finally, it is by being able to project into scenarios several years or ten years ahead that the most structuring decisions can be taken (by looking, for example, at the evolution of water availability with climate deregulation).
This makes it difficult to make decisions without access to data. Some départements, for example, don’t even know how much of their territory is irrigated. Management models (e.g. setting a withdrawal volume based on a dry five-year period) in fact make the assumption that we have no knowledge of the water resource. This knowledge is available, however, if we monitor abstractions on a regular basis, so that we can make ongoing, concerted trade-offs and seek margins for manoeuvre. Knowledge of the hydrological functioning of our watershed and the ability to model its status and future evolution are essential if we are to imagine integrated water resource management. Data can thus sometimes be seen as a factor of appeasement when there are controversies and tensions.
This will certainly also involve institutional modernization of certain water-sharing rules, with some structures not complying with current rules. This is the case, for example, with old ASAs (authorized syndical associations) where old Napoleonic water rights still reign. Some water rights are still linked to the construction of canals, at a time when there were also local arrangements based on land ownership. These water rights were defined independently of the physical reality of the territory. Some farmers would also have reference quotas, on the pretext that they were the first to have access to irrigation, without questioning these references in the light of current developments.
There is no universal model for water. We must be careful not to fall into an over-powerful rationalization of water. An over-objectification of the quantitative aspects of water sometimes tends to desensitize and depoliticize water debates. Current thinking is very much focused on quantitative water management, with debates on abstraction levels (volume studies) and the idea that everything available can still be abstracted. This trend towards depoliticization can also be seen in the delegation of water management to public authorities and the transfer of water management powers to larger administrative scales.
Mega basins and substitution reserves
Recent events suggest that a form of water war, or at least water appropriation, has begun. Echoing the battles waged at the Sivens dam in the Tarn region in 2014 (which resulted in the death of activist Rémi Fraisse), debates over water use have been revived in the wake of the controversial Saintes Solines mega-pool in the Deux Sèvres region in 2023. The government deployed an armed force of over 3,000 police officers to defend a project to build a mega-basin – or rather, as things stand, an empty hole. After a weekend-long outburst of violence by the police and the BRAV-M quad brigade, damage was caused on both sides, and two activists left the demonstration in a coma (the government having given orders not to let the ambulance intervene). Despite the overwhelming images and videos of violence, the government is sticking to its guns, and doesn’t even dare to take responsibility for the inhumanity of the forces involved (the BRAV-M would obviously never have beaten the activists with their truncheons, despite the irrefutable evidence that has been circulating on the networks), and the totally unbalanced violent responses of the forces of law and order (LBD, disencerclement grenades, Brav-M) in relation to the violence of the activists. Worse still, the government has pushed for the collective dissolution of Les Soulèvements de la Terre in June 2023.
In France, apart from the large dams that also serve other purposes (power generation, drinking water), many reservoirs are used primarily for irrigation. While the principle is simple – to create water reservoirs – there are many different ways of doing this. The number of such reservoirs in France and the total volume stored in them are not precisely known, nor is the proportion actually used for agricultural irrigation. We only know that there are several tens of thousands of them, storing several hundred million m³. The absence of collective management of their filling, maintenance, use and emptying conditions, and hence the lack of control over their impact on the environment, as well as their exclusively irrigation-oriented purpose, mean that water agencies do not subsidize them. What’s more, a significant proportion of them are no longer used, or are only partially used. Remobilizing these abandoned or under-utilized reservoirs for irrigation raises technical, financial and legal issues that make it hypothetical under current law.
Reservoir projects are most often led by farmer groups, ensuring collective management of water resources on a sub-basin scale, rather than individual management with no coordination between farmers.
Mega reservoirs (also known as surrogate reservoirs) are huge structures that do not fill up by tapping the flow of water, but only by pumping water from rivers and groundwater. Mega-reservoirs differ from conventional reservoirs or dam reservoirs in that they are neither located on a river nor on a landform. The reservoir must therefore be excavated and dammed all around, giving it the shape of a basin. These mega-basins are intended to play the role of water insurance for agricultural producers, securing a resource that may not be available during the summer months. Unlike other storage infrastructures, mega-basins could be subsidized up to 70% by the French Water Agency. A dynamic map of existing basins is available here
The Coopérative de l’Eau des Deux Sèvres’s project for surrogate reservoirs aims to respond to the planned reduction in volumes that can be drawn from the environment in spring and summer, by replacing some of these summer withdrawals with winter pumping and storage of this water in reservoirs for use in spring and summer.
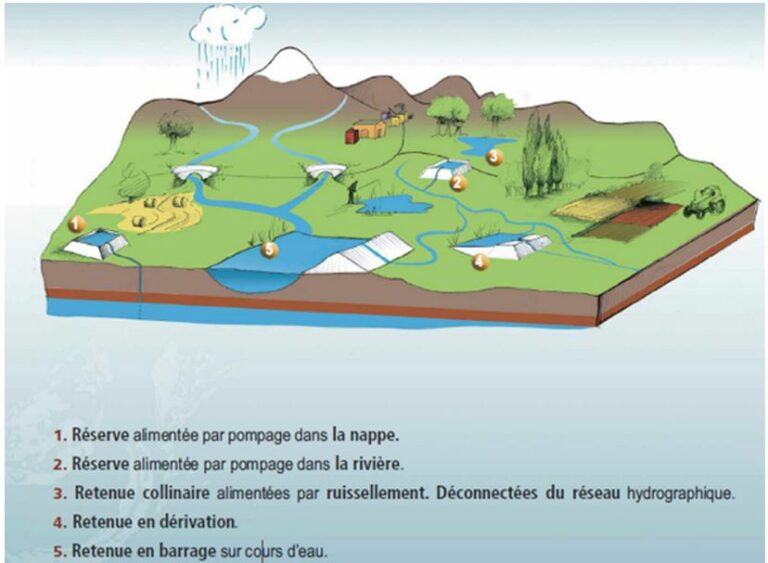
Figure 40. Forms of water catchment. The Saintes Solines mega-basin is a type 1 basin. Note: Dam lakes do not fill up by storing the rain that falls. The sum of these withdrawals can then contribute to increasing the duration of the drought. For this reason, we speak of “anthropogenic” drought.
The case of Saintes Solines received particular media attention because, according to information relayed by numerous media, a BRGM (Bureau de Recherches Géologiques et Minières – Geological and Mining Research Bureau) study had demonstrated the harmlessness of this replacement reservoir on the environment. The quality of the report was then criticized in several quarters, as it was in fact a document produced by BRGM’s commercial studies section in response to a private contract from a local irrigators’ cooperative in the Marais Poitevin. BRGM had then proposed a document that answered only the questions asked (and the scenarios requested) by the cooperative, and which should never have been considered as a reference document on the installation of the so-called mega-basin. In fact, BRGM was forced to issue an emergency press release – rather well written – following the growing excitement surrounding the subject.
The initial BRGM (Bureau de Recherches Géologiques et Minières – Geological and Mining Research Bureau) report only provided hydro-geological results on the water table and associated rivers, with methodological limitations highlighted by its client (notably the resolution of the simulation model at the kilometre grid, which could not be used to deduce anything at finer grids). It also showed that the threshold values for water table elevation – set by the cooperative – were lower than the average for the last five years (see the section of the dossier on low-water and biological flows), emphasizing that the thresholds were there to guarantee that the basins would be filled, but not to ensure that the rivers would flow and that the water tables would be recharged.
The cooperative should have focused on complementary issues:
- Taking into account a recent climatic period, or even a period in the future. In fact, the study did not consider a climatic period in the past between 2000 and 2010, far removed from current climatic conditions. This period would nevertheless have enabled us to assess what would have happened if the replacement reserves had been put in place during the years 2000-2011, bearing in mind that these years are representative of contrasting weather situations (wet and dry years).
- Taking into account the financial and energy costs of pumping, and the kilometers of supply networks to be built.
- Taking into account the impact on the reservoir (evaporation, development of algae and bacteria, etc.).
- Taking into account the complexity of watercourses. The sinuosity and multiplicity of watercourses is an essential factor in infiltration and discharge of the water table.
Mega-basins could be the source of a double disconnection, both spatial and temporal. Temporal disconnection, because pumping and filling (rainfall) do not necessarily follow one another. Add to this the sometimes very long latency periods, which mean that it may take several years for the water table to return to low water. And a spatial disconnect, because the water tables are not necessarily recharged where they are most needed and where the water is actually pumped.
The storage infrastructure model is not new, however, and some countries bordering France – Spain, for example – have already paid the price. This model is not resistant to long periods of drought. With more than 1,200 reservoirs and artificial lakes, the Iberian peninsula is Europe’s champion in terms of water reservoirs, but this model, which is mainly focused on supply, is actually quite limited. Spain has based its economic development on water, implementing a policy based on the construction of major infrastructures (canals, dams, etc.) to mobilize available water resources and transfer part of the water from the Atlantic basins to the Mediterranean basins in deficit. According to the Scientific Council of the Rhône-Méditerranée Basin Committee, a model focused on supply is the cause of territorial competition between traditional farmers and new irrigators, between agricultural activity and other uses, of mistrust between regions over the sharing of water and, among other things, of a dramatic deterioration in river water quality.
The national plan for adaptation to climate change (PNACC2) stresses the importance of implementing winter water storage projects, where useful and sustainable, on the basis of the best possible knowledge, reconciling activities with each other and with the preservation of the environment, in particular aquatic ecosystems, with priority given to areas in quantitative imbalance or likely to be so in the near future (Action NAT-2).
Winter groundwater abstraction assumes sufficient recharge over the period, which is relatively difficult to predict given the effects of climate change and, in particular, the inter-annual variability of precipitation. A second point of attention relates to the impact of winter abstractions on the hydrological regime and aquatic environments, including the ecological functioning of estuaries and coastal ecosystems. Whatever they may be and if they are implemented, water abstractions must also be taken into account in relation to irrigation practices, to ensure that the various low-water flows are maintained (biological, ecological, etc. – I refer you to the corresponding section of the dossier). In particular, it is important to consider the extent to which planned advances in irrigation calendars could compensate for the earlier and more severe low-water periods predicted by hydrologists as a result of climate change, and what adaptations to irrigation practices should be put in place to amplify the positive effects of phenological advancement (sowing dates, choice of earlier varieties) and thus limit withdrawals during low-water periods.
It’s important to remember, however, that reservoirs can also have positive impacts. The ones most often put forward revolve around the economic development of a region and the impact on the economic players in the basins concerned, but we can also find some in connection with the biodiversity present on site.
Mega-basins are particularly divisive, because for many, as proposed, mega-basins seem to benefit only a small number of farmers, often representative of an agricultural model that has reached its limits. It remains unclear whether the charters that are supposed to be signed as part of the basin program are sufficiently ambitious, and above all whether they are subject to obligation and future auditing by the farmers concerned. On the contrary, the main message seems to be a desire to secure access to a given volume of water – calculated without any awareness of natural reserves – to the detriment of all the region’s agriculture and young farmers wanting to set up in the area. Some even speak of a generalized theft of water tables.
The work put forward often takes a hydrological angle, focusing on the environment. And the criticisms are often numerous: impacts within the reservoir (evaporation, sediment traps, phosphorus storage, risks of eutrophication, possible invasive species), impacts downstream (modification of the hydrological regime and solid transport, reduction in mean annual flow, etc.) and ecological impacts (disruption of ecological continuity) depending on the location of the reservoir, its characteristics and its management method.
Yet it’s very important to get everyone around the table, including hydrologists, hydro-geologists, agronomists, climatologists and farmers. The first thing to note is that all French regions are different. There are, for example, the Mediterranean regions, which are structurally in annual water deficit, and south-west France, where the annual deficit is not systematic, and where the IPCC climate scenarios do not foresee it becoming so in the near future. There may be a case for using the topography of a watershed to recover runoff where water is lacking, provided that the main watercourse is not dried out.
The debate on mega-basins cannot afford the luxury of overshadowing agricultural issues. The idea here is not to demonize farmers, especially as some farmers in the Sainte Solines area have made enormous efforts to move towards more resilient practices or to break away from the dominant agricultural model, even if the vise is sometimes so tight that it’s almost impossible to break away.
So the debate is not so much about the use of water, but its misuse. Irrigation in itself is not a problem, because certain crops (e.g. horticulture), especially in certain regions of France, are simply impossible without water. In the final analysis, opponents of reservoirs question the usefulness of spending large sums of public money to build infrastructures that benefit only a few water-using farmers, which in their view constitutes an unacceptable attack on water’s status as a public good. But there is no scientific basis for a blanket disqualification of water storage. It is a case-by-case analysis, through procedures that are already very demanding, that must determine whether it is possible, territory by territory, to create new reserves. We also need to avoid the rebound effect whereby secure access to a water resource increases water use and system dependency.
The risk cannot be borne by farmers alone
It’s easy enough from the outside to give farmers advice on how best to manage water. Yet they are the ones taking the risk and potentially gambling all their savings with the decisions they make. Water management has a very stressful side, and is a huge responsibility for farmers – especially when employees and family are involved. Consideration of more water-efficient crops or production methods is rare or sometimes very difficult, due to the lack of agronomic alternatives to existing crops, with the risk of the abandonment or even disappearance of agriculture in the area concerned. On the other hand, since drought decrees are subject to change, farmers do not know in advance whether they will be banned from irrigating.
It’s this notion of risk sharing (in the same way as there is value sharing) that we need to work on with, for example :
- The mobilization of economic players in the sector to design and implement agri-chains based on more water-efficient crops. Farmers are confronted with the only supply chains in place on their territory, and sometimes find themselves in a locked-in system linked to the structuring of existing agricultural systems (for example, compulsory voluntary contributions – usually reinforce existing supply chains). Commodity chains must not abandon farmers, and must bear their share of the risk, by avoiding, for example, relocating their processing plants or their sources of supply for contract crops if water limitations in France become more severe. Sectors need to transform and restructure: business models, investment policies, relocation of certain facilities, downsizing of industrial facilities…
- The mobilization of public aid through the generalization of irrigation management, the purchase of equipment, the deployment of irrigation optimization tools, and calls for projects for new, more water-efficient sectors (soybean, sunflower, legumes, etc.).
- The mobilization of the insurance sector with, for example, the obligation to set up climate conversion contracts to support farmers’ risk-taking. Lack of knowledge about these alternative crops, as well as the uncertainty associated with their implementation, all contribute to slowing down change.
- The mobilization of consumers (or rather, consumer-actors), with their ability to support, or even instigate, this transformation through their purchasing preferences or changes in diet.
A Common Agricultural Policy (CAP) that doesn’t do enough to encourage more rational use of water
According to the European Environment Agency (EEA), water consumption for agricultural purposes in the European Union has fallen by 28% since 1990, while excess nitrogen and nitrate concentrations in rivers have dropped by 10% and 20% respectively since 2000. However, further improvements in the 2010s have been modest, and the pressures that continue to be exerted are far from sustainable.
CAP direct payments do not really encourage more rational use of water. Currently, payments under the Single Area Payment Scheme and the Basic Payment Scheme have a neutral effect on irrigation: they provide no incentive to use water rationally, nor to irrigate or use more water. CAP performance indicators tend to focus, for example, on the number of hectares covered by water-friendly environmental management, rather than on the volume of water used for irrigation in agriculture, or the rate of nitrate pollution. ). CAP subsidies favor field drainage over water retention. Drained peatlands may qualify for income support under the 2014-2020 CAP, while cultivated wet peatlands are sometimes considered ineligible during inspections.
Neither these two direct payment schemes nor the green payment scheme impose any obligations on farmers with regard to sustainable water use. The green payment scheme may, however, have indirect positive effects through the obligation placed on farmers to preserve permanent grassland (which, unlike arable land, is not normally irrigated).
The second pillar of the CAP provides funding for projects and practices designed to improve the sustainable use of water under rural development programs. For example, it finances water retention measures, wastewater treatment facilities and projects to improve the efficiency of irrigation systems. Successful projects of this kind have contributed to better water management at local or regional level, and thus to the protection of water resources at this level. However, other types of project can also be financed by the second pillar of the CAP, even though they are likely to increase demand for water. Examples include the extension of irrigable areas or new irrigation projects.
The policies of member states are not always aligned with EU water policy. Catchment authorization systems and water pricing mechanisms involve numerous derogations when it comes to the use of water for agricultural purposes. Few CAP schemes make payments conditional on compliance with strict requirements for sustainable water use. Cross-compliance, a mechanism that can lead to – generally small – reductions in subsidies paid to farmers in the event of proven non-compliance with certain requirements, promotes sustainable water use, but does not apply to all CAP subsidies, nor to all farmers.
All in all, the CAP has not led to water savings, nor to any significant improvement in surface water quality. In a special report published in 2021, the French “Cour des Comptes” concluded that EU funding of irrigation projects was not accompanied by sufficient safeguards for sustainable water use.
The new CAP 2023-2027 opens up an opportunity to make progress on the subject of water, because the implementation of strategic plans (NSP – national strategic plans) and the disbursement of sums from the European budget are conditional on the monitoring and achievement of objectives. However, the Environmental Authority notes that the French strategic plan’s resources in this area have been significantly reduced compared with the previous CAP period. The Authority suggests that the trajectory mapped out by the future NSP will not match that of the national low-carbon strategy (SNBC), the biodiversity plan or the Water Framework Directive by 2030. The Environmental Authority notes that the inclusion of a parameter related to the size of the territory concerned smoothes out the level of challenge for certain issues that are nevertheless particularly acute locally, notably water. Nor does strict application of national and European regulations appear to be a sufficient solution for improving the situation (e.g., compliance with reserved flows during low-water periods, or with the objectives of the Water Framework Directive to achieve good river status by 2027).
The French recovery plan is worth €100 billion, of which €40 billion is financed by the European Union through the “Next Generation EU” program. Despite this, 85% of the measures are assessed as neutral with regard to water resources, based on a self-assessment in the form of a simple affirmative statement in the plan, often lacking more analytical elements to justify this position (Institute for European Environmental Policy, 2022). Of the dozen or so measures considered to have a positive impact on water, around half appear to be overly optimistic in their assessment. For example, the “green budget” measure in Strand 3 is considered positive for water on the grounds that the corresponding expenditure is classified according to six environmental classes.
The various European instruments available to preserve water as a natural resource and improve its management are present and, on first analysis, generally sufficient to enable progress. Despite this, these instruments lack coordination between them, and even risk certain contradictions, either due to a lack of prioritization of the water issue (in recovery plans in particular), or due to the stacking of measures with divergent vocations (between the 1st pillar of the CAP and the 2nd, for example).
The CGEDD/CGAEER special report also proposed, on the subject of water, to :
- make rural development support for investment in irrigation conditional on the implementation of policies promoting the sustainable use of water in the Member States;
- link all CAP payments to farmers, including those made via the Common Market Organization, to explicit environmental requirements for sustainable water use, notably through cross-compliance;
- require safeguards to be put in place to prevent the unsustainable use of water for crops financed by optional coupled support.
Nor are the timetables for the recovery plan and the CAP very consistent. On the one hand, the stimulus package called for rapid action on the part of governments. On the other hand, the CAP was delayed by two years, so that strategic plans could be drawn up by the Member States in line with Green Deal objectives. However, this timetable prevents the water-related investments contained in the recovery plans from being aligned with the hydraulic needs of agriculture as derived from the CAP’s strategic plans, since they have not yet been finalized.
Political news slowly taking an interest in the subject
For some, France has its share of water management paradoxes. Despite its supposed abundance of water, France is already facing severe water insecurity and the promise of a climate that is becoming Spanish, with extreme soil drought. France stores, irrigates and invests little in irrigation infrastructure, while massively importing virtual water from neighboring countries. Our neighbors are planning and investing, but are overexploiting their resources, which raises fundamental questions about our food dependency, which is only likely to increase. If the countries that supply us with virtual water also run out of water, it’s the beginning of a global catastrophe.
In May 2021, the government made a big splash by launching the “Varenne agricole de l’eau et de l’adaptation au changement climatique” (agricultural exhibition on water and adaptation to climate change), which followed on from the Assises de l’eau (Water Conferences) of 2019. These events led to the definition of a series of actions, including the introduction of collective management and water-sharing rules, with the aim of reducing withdrawals linked to all uses (domestic, industrial and agricultural) by 10% by 2025 and 25% by 2035. In early 2023, the government launched a National Water Plan in response to the severe crises and droughts of the past few years. No fewer than fifty measures were proposed, some of them directly linked to the agricultural sector (see below). However, we are surprised to see that the targets for reducing water abstraction have already been pushed back since the French Water Conferences, since the French National Water Plan of 2023 only mentions a 10% reduction in abstraction by 2030, compared with the almost 25% announced at the last French Water Conferences. While this remains an ambitious target, it may also reflect a desire not to move too quickly…
Some key elements of the Water Plan directly related to the agricultural sector:
- Sobriety of use for all sectors (with a target of a 10% reduction in abstractions by 2030) with better territorial planning and better measurement of the state of resources.
- For farmers: an additional €30 million per year will be earmarked to support water-saving farming practices (emergence of low-water consumption sectors, drip irrigation, etc.).
- The installation of meters with remote transmission of volumes abstracted will be made compulsory for all major abstractions (corresponding to environmental authorization thresholds).
- Optimize resource availability by reducing leakage (on the demand side), enhancing the use of non-conventional water (on the supply side) and improving storage in soil, structures and groundwater (on the supply side).
- The preservation of wetlands will be strengthened with an additional €50m/year in payments for ecosystem services, and the Conservatoire du Littoral will consolidate its land acquisition strategy.
- A €30 million/year agricultural hydraulic investment fund will be set up to remobilize and modernize existing facilities (cleaning out reservoirs, maintaining canals, etc.) and develop new projects that respect the balance of uses and ecosystems.
- Preserve water quality and restore healthy, functional ecosystems by preventing environmental pollution and developing nature-based solutions to restore water cycles.
- Agri-environmental and climate measures (MAEC) and organic farming subsidies will be upgraded in catchment areas, to the tune of 50M€/year. Experimental payments for environmental services (PES) will be maintained until the next CAP, at a level of 30M€/year.
- When it comes to setting up new farmers in water catchment areas, we’ll be promoting agro-ecological and organic farming projects. This ambition will be pursued as part of the Pacte consultation process, and as part of the Agricultural Orientation and Future Law.
- Implement regulatory and consultation tools to improve water management, by reworking the governance of water management, investing in research and innovation, and using economic instruments (water pricing, financing of water resource management).
The government is currently working on an agricultural policy pact, which we hope will focus on climate change adaptation and water-related issues – as no national agricultural document has yet given sufficient consideration to these issues. Some national and regional agricultural planning and programming documents do, however, take climate change into account. These are mainly regional rural development programs and national and regional agricultural and rural development programs.
Finally, let’s mention the PEPR (Priority Research Programs and Equipment) “One Water” launched in 2022 by the government to think about water management in a context of global change, where pressures on water are increasing.
Everyone needs to be trained – keep alerting
All stakeholders in the region need a minimum level of hydrological literacy to understand the main principles at work. We have to assume that we don’t understand the water cycles at work. For some authors, the exclusion of human activity from the water cycles classically presented is not intended to simplify these cycles for the general public (Abott et al., 2019). On the contrary, it would be an omission that would render the hydrological cycle incomprehensible in the Anthropocene era. Indeed, it is no longer possible to understand the spatio-temporal distribution of water quantity and quality on Earth without taking human activity into account. Human alterations to water, land and climate have so altered the water cycle that model simulations based solely on natural dynamics can no longer reliably predict groundwater levels, droughts, floods or precipitation. Important messages must also focus on the quantity of water available, or rather the small amount of water that can actually be used by humans and ecosystems in relation to the total water available on the planet (see section on orders of magnitude at the start of this dossier).
The general public is already relatively uninformed about water-related issues and policies. The effective implementation of water management policies presupposes public support, but the information disseminated is generally very, if not too, technical. Nor does the territorial scale of water management correspond to the catchment area of the general public, who are not very involved in public debates and consultations on these subjects (even if the demonstrations at Saintes Solines have rekindled the debate on water).
This is understandable, given the complexity of the organization of players in the region (Fig. 33). Water management bodies and tools (Water Agency, Watershed Comity, Local Water Commission…) are generally little known and considered particularly confusing. The scale of the large hydrographic basin, and therefore of the SAGE/SDAGE, has no reality for the population.
Farmers, too, obviously need to develop their skills in this area, and it would be conceivable for farmers, on a model similar to the EcoPhyto plan’s Certiphyto certification, to be able to access a Certirrig-type certification to attest to a level of training and knowledge that ensures the use of water-saving equipment and practices. Does a better-informed farmer pay more attention? One might be tempted to think so, at least in areas where there are water shortages. Of course, raising awareness is not a sufficient condition for taking action, but it is an absolute prerequisite. Some farmers are also very helpless, caught up in a system that is beyond them. Disseminating knowledge and setting up large-scale networks of experimental/demonstrator pilot farms is important. Farmers are deeply rooted in the reality of the current crop year (weather forecast), with a vision often restricted to the last two or three crop years. As a result, it is often useful to recall past climatic events in terms of chronology and magnitude over a period of several decades. The notion of adaptation, at the heart of today’s major issues, needs to be widely popularized and explained.
The value and very notion of the existence of resources needs to be questioned. We need to reinvent the social contract.
In conclusion
There’s an abundance of digital offerings on the subject of water in agriculture. From tools for measuring and observing water in the various plant-soil-atmosphere compartments, to modelling and decision-making tools for irrigation management, to infrastructures for making data available to users and decision-makers, digital technologies are one of the strings to our bow when it comes to thinking about dynamic multi-use management of water resources, for which the pressures are only going to intensify.
Once again, irrigated areas will account for just 7% of France’s usable agricultural area by 2020. And irrigation is still the main focus of digital agricultural technologies. Current tools are still based on the agriculture we know, and this raises the question of the potential for irrigation management in agricultural systems that evolve with different water dynamics. To avoid this focus on irrigation, we need to reverse the priorities, i.e. start by adapting rain-fed agriculture to ongoing climate deregulation, and only consider irrigation as a last resort. Progress in rain-fed agriculture will certainly be even more decisive than that of irrigated crops.
Digital tools, as suppliers of potentially objective data, can act as a justice of the peace and a basis for concerted collegial reflection. Nevertheless, the desire to count and measure everything can obscure the real intentions behind the monitoring and fine-tuning of water resources. Measuring and quantifying an increasingly limited resource is obviously laudable, but it must be done in the service of a transition towards a responsible water policy, focused on demand, and in consultation with all stakeholders.
In their 2020 report on “Climate Change, Water and Agriculture”, the French General Councils for Agriculture and the Environment called for water to be considered from a multi-annual perspective, for agricultural issues to be territorialised and made coherent with local issues (and therefore with regional planning documents), and for water governance to be reworked, with clearly articulated decision-making bodies.
The historical model of water management is still one of unlimited management, centered on a strategy of supply, with the desire to provide water to users as long as they need it. A policy based on demand must take over, and it seems that agriculture is not yet prepared for this. The economic stakes are high, and the transitions can be particularly onerous. Yet there are other ways of thinking about water, its management, sharing and governance. We need to move away from a simple logic of water efficiency in agriculture to revisit broader concepts of enhanced water resource management.
Finally, we have to accept the fact that we are still very little acculturated to the subject of water and its complexity. We don’t know much about water cycles and how humans are strongly involved in them. We still lack knowledge of plant ecophysiology and how plants regulate their water flows. And although water management must also be thought of on a local and operational scale, we must not forget that water issues go far beyond our borders. New concepts such as atmospheric basins remind us of this.
Bibliography to complement the interviews
Abbott, B., et al. (2019). A water cycle for the Anthropocene. Hydrological Processes, 33, 3046-3052
Abbott, B., et al. (201). Human domination of the global water cycle absent from depictions and perceptions. Nature Geosciences, 12
Abiodum Abioye, E., et al. (2020). A review on monitoring and advanced control strategies for precision irrigation. Computers and Electronics in Agriculture, 173
ADEME (2010). Climator. Climate change, agriculture and forestry in France: simulations of impacts on the main species.
Ademe (2020). Life AgriAdapt. Adapting agriculture to climate change. Characterizing the vulnerability of farms to cliimatic change in order to identify sustainable adaptation actions. Report – 44p.
Ademe (2022). Transitions 2050. Adaptation to climate change: transport, agriculture, forests, industries, buildings. Report – 4pages.
Loire-Bretagne Water Agency (2022). Analyses Hydrologie – Milieux – Usage – Climat (HMUC). Guide et recommandations méthodologiques. Report -64p
Agence de l’eau Rhône Méditérrannée Corse (2022). Social and territorial utility. An opportunity for major water cycle projects. Report – 20p
Ahmad, U., et al. (2021). A Review of Crop Water Stress Assessment Using Remote Sensing. Remote Sensing, 13
Association nationale des élus de bassins -ANEB (2022). Le livre bleu – l’eau en commun, 60 p.
Environmental Authority (2021). Deliberate opinion of the Environmental Authority on the National Strategic Plan for the Common Agricultural Policy 2023-2027
Berger, M., & Finkbeiner, M. (2012). Methodological Challenges in Volumetric and Impact-Oriented Water Footprints. Journal of Industrial Ecology
Berger, M., Pfister, S., Motoshita, M. (2016). Water Footprinting in Life Cycle Assessment – How to Count the Drops and Assess the Impacts? Chapter 3 – LCA Compendium – The Complete World of Life Cycle Assessment
BRGM (2022). Simulation of the Coopérative de l’eau des Deux-Sèvres 2021 substituion reserve project. Final report – 143p
CGAAEER (2022). Parangonnage sur les techniques et pratiques innovantes de gestion de l’eau en agriculture. Report – 90p
CGEDD, CGAAER (2020). Climate change, water, agriculture: what trajectories to 2050? Report – 333 p.
CNDP (2022). La participation sur l’eau : Bilan de 25 ans de débats publics et concertations. Report – 6p.
Collard, A-L., et al. (2019). A “smart” meter to measure water uses: the entry of a new knowledge. Développement durable et territoires, vol 10.
Comité 21 (2022). Sector guide to adaptation to climate change
European Court of Auditors (2022). The CAP and the sustainable use of water in agriculture: funds more likely to encourage more consumption than better consumption. Special report – 63 p.
Cour des Comptes (2023). Rapport Public Annuel. Decentralization, 40 years on.
De Ponti, T., et al. (2012). The crop yield gap between organic and conventional agriculture. Agricultural Ecosystems, 108, 1-9
Ezenne, G.I. et al. (2019). Current and potential capabilities of UAS for crop water productivity in precision agriculture. Agricultural Water Management, 218, 158-164
FAO (2015). Yield gap analysis of field crops: Methods and case studies. FAO Water Reports. 41
FNCCR (2022). Encouraging water savings. Contribution of behavioral sciences. Water Saving Club recommendation sheets
France Stratégie (2023). Cost of inaction on climate change in France: what do we know? Working paper
Galabert (2022). Understanding hydrological cycles and cultivating water to restore soil fertility and care for the climate, Documentation ISI – Intercultural Initiatives and Solutions, Version 2
Gong, X.Y., et al. (2010). Tradeoffs between nitrogen- and wate-use efficiency in dominant species of the semiarid steppe of Inner Mongolia. Plant Soil
Inrae (2022). Society – Will agriculture run out of water? Ressources. N°2
Institute for European Environmental Policy (2022). Water management in agriculture. What’s in the European recovery plans? Report – 42 p
IPCC (2022). AR6 Climate Change: Impacts, Adaptation and Vulnerability. Chapter 13
Loubier et al, (2013). Is irrigation declining in France? First lessons from the 2010 agricultural census. Sciences Eaux et Territoires.
Marechal, J-C., & Rouillard, J. (2020). Groundwater in France: resources, use and management issues. Sustainable Groundwater Management. Chapter 2
Mounier, B. and Uso, T. (2022). La financiarisation de l’eau, menace fantasmée ou réelle? Attac, 33
Hatfield, J.L., & Dold, C. (2019). Water-Use Efficiency: Advances and Challenges in a Changing Climate. Frontiers in Plant Science, 103
Petit, O. (2004). The new resource economy and water markets: an ideological perspective? VertigO – La revue en sciences de l’environnement, vol 5
Plateforme des bonnes pratiques pour l’eau du bassin Adour Garonne (2023). Improving irrigation water efficiency through management.
Reghezza, M. and Habets, F. (2022). Are mega-basins viable solutions to drought? Bon Pote
Rockstrom, J., et al. (2023). Why we need a new economics of water as a common good. Nature
Schneider, L. et al. (2021). Sharing irrigation water in watersheds: uses and interests of quotas. 15th Journées de Recherche en Sciences Sociales (JRSS).
Senate (2022). Rapport d’information fait au nom de la délégation sénatoriale à la prospective sur l’avenir de l’eau. Report – 166 p
Serra-Wittling, C., & Molle, B. (2017). Evaluation of plot-based water savings achievable by modernizing irrigation systems. Report – 150p.
Serra-Wittling, C., & Ruelle, P. (2022). Guide pratique de l’irrigation – 4th edition. Editions Quae
Van Ittersum, M.K., et al. (2013). Yield gap analysis with local to global relevance – A review. Field Crops Research, 143, 4-17.
Yao et al (2023). Satellites reveal widespread decline in global lake water storage. Science. Global Hydrology, vol 380 World Meteorological Organization (2022). State of Global Water Resources 2021. N° 1308.
Interviewees
Name | Structure |
Maïder ARREGUI | BRL exploitation |
Nicolas BAGHDADI | INRAE |
Gilles BELAUD | UMR G-Eau |
François BRUN | ACTA |
Denis BOISGONTIER | Cap 2020 |
Anne-Laure COLLARD | UMR G-Eau |
Rémi DECLERCQ | Ecofilae |
Valérie DEMAREZ | CESBIO |
Sophie GENDRE | Arvalis |
Jean-Christophe MARECHAL | UMR G-Eau |
Bruno MOLLE | UMR G-Eau |
Julien LECONTE | Water Chair |
Marine LEMOIGNE & Marjorie DABRIN | Vegetal Signals |
Sébastien LOUBIER | UMR G-Eau |
Charlotte PRADINAUD | ELSA Chair |
Alice RACTMADOUX | SCP |
Dominique ROLLIN | Ex-CGEDD |
Thibaut SCHOLASCH | Fruition Sciences |
Vincent SIMONNEAUX | CESBIO |
Dominique THERIEZ | Aquasys |
Arnault TRAC | Hydroclimate |
Benoit WIBAUX & Marion VIGUIER | Platform of best practices for water in the Adour Garonne basin |
Serge ZAKA | ITK |